The transition to Holocene interglacial conditions
- This page is part of the topic The last million years
The transition from the LGM (beginning about 21 ka BP) to the present interglacial period (Termination I) was the last major global climate change event. The Northern Hemisphere experienced dramatic changes during the deglaciation starting with an abrupt warming (the Bølling-Allerød) at 14.7 ka B2K (i.e. before AD 2000; an ice core dating convention introduced by Rasmussen et al. (2006[1]) as an alternative to the conventional Before Present (BP) where ‘Present‘ is 1950), followed by a return to colder conditions (the Younger Dryas episode) and a final rapid warming leading to the Holocene at 11.7 ka B2K (Rasmussen et al., 2006[1]). In contrast, long climate records extending back to 800 ka BP, recently obtained from deep Antarctic ice cores, have revealed the exceptional character of this last termination: neither the Antarctic temperature proxies nor the methane records capture such abrupt changes during the earlier terminations (Spahni et al., 2005[2]; Jouzel et al., 2007[3]).
Because it is the most recent transition between a glacial and an interglacial period, Termination I can be examined with high resolution data and a robust age control (EPICA, 2006[4]; Rasmussen et al., 2006[1]). Ice core records can be synchronised either regionally using aerosol tracers (e.g. dust particles including calcium from continental aerosols, or sulphate from volcanism) (Severi et al., 2007[5]) or globally using well-mixed atmospheric gas records (such as CH4 or o18O of O2) (Blunier et al., 1997[6]; Morgan et al., 2002[7]; Blunier et al.. 2007). The accuracy of the Greenland ice core layer-counted age scale GICC05 is between 100 and 200 years over the last transition (Rasmussen et al., 2006[1]). The error on the transfer of this age scale to Antarctic ice cores using CH4 synchronisation is estimated to be at most 250 years for the Younger Dryas period (the abrupt cooling interrupting the Northern Hemisphere deglaciation). Since the pioneer works of the 1960s, Greenland and Antarctic ice core records have been used to determine the magnitude of climatic and environmental changes and the precise sequence of events during the last major climate transition.
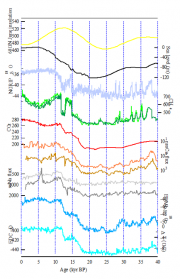
Termination I is now documented at a high resolution in more than 10 Antarctic ice cores located in both East and West Antarctica (Figure 3.14). The beginning of the Holocene at ~11.7 ka BP (Rasmussen et al., 2006[1]) can be found at various depths depending mainly on the accumulation rate and the ice flow at different locations: ranging from ~270 m at Vostok to ~1120 m at Law Dome; note that it is found even deeper in Greenland (~1490 m at NorthGRIP, ~1620 m at GRIP). The full length of the transition varies between ~20 m at Taylor Dome or Law Dome, 100 m at Siple Dome, ~135 m at Vostok, ~155 m at EPICA Dome C, ~290 m at Byrd and up to 300 m at EPICA Dronning Maud Land. Climate records of Termination I from different Antarctic ice cores can therefore offer varying temporal resolutions. The records are affected by a strong thinning at coastal locations and may be partly affected by changes in ice sheet elevation and ice origin from upstream areas at inland locations, especially those not located on domes. The quality of the ice core samples may be affected when the transition is located in the brittle zone – cores taken from the range approximately 400 to 1,000 m depth, often described as brittle, can be easily damaged by drilling and handling.
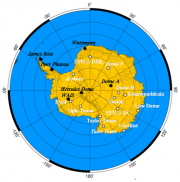
Figure 3.15 shows selected examples of Antarctic ice core records of Termination I. These records offer the potential to compare local (Antarctic site temperature / accumulation), regional (sea salt and marine biogenic sulphur concentrations), hemispheric (concentration of different Antarctic dust fractions) and global (greenhouse gas concentrations in the atmosphere) climate and environmental parameters which are described in more detail below. The stable isotopic composition of ice (aD or 18O) is classically used to quantify past Antarctic surface air temperature changes. The strong linear relationship observed between snowfall isotopic composition and temperature (Masson-Delmotte et al., 2008[24]) is caused by the progressive cooling and distillation of air masses along their trajectories from oceanic moisture sources to inland Antarctica. General atmospheric circulation models have shown that this relationship remains stable in central Antarctica at the glacial-interglacial scale, leading to uncertainties of 20-30% on reconstructed temperatures (Jouzel et al., 2003[25]). Temperature estimates based on stable isotope records may be biased by deposition effects related to the seasonality of snowfall. Climate models suggest that this effect remains limited inland in Antarctica (Werner et al., 2001[26]). However, past changes in evaporation conditions may affect Antarctic snowfall isotopic composition. The deuterium excess parameter (d=fD-8-18O), mainly affected by a kinetic fractionation effect, has been used to quantify past changes in moisture source and site temperatures more precisely (Stenni et al., 2001[27]; Vimeux et al., 2002[28]). The moisture source temperature is an integrated quantity that may correspond to either changes in sea surface temperature in the dominant evaporation areas or to geographical changes of the main moisture origin with time.
Most Antarctic ice cores exhibit comparable magnitudes of glacial-interglacial water stable isotope changes, with any major departure likely to be due to changes in ice sheet elevation. The magnitude of glacial-interglacial temperature change in central Antarctica has been estimated to be 9°C with an uncertainty of 2°C (Stenni et al., 2001[27]). In central Antarctica, the coherence between the various temperature records based on stable isotopes is very high (within 1°C) (Watanabe et al., 2003[22]). Based on stable isotopes, Antarctic temperatures appear very constant during the period from 19 to 23 ka BP without a clear “glacial maximum” (i.e. temperature minimum). A first Antarctic warming phase associated with Termination 1 is identified as starting at about 19 ka BP in central Antarctica. The warming is interrupted by a 1.5°C cooling from 14 to 12.5 ka BP, the “Antarctic Cold Reversal” (Jouzel et al., 1995[18]). The second warming phase into the early Holocene is the fastest temperature rise detected in the 800 ka EPICA Dome C record, with a pacing of ~4°C per 1,000 years (Masson-Delmotte et al., 2006[29]). Intergovernmental Panel on Climate Change (IPCC) Assessment Report 4 coupled ocean-atmosphere-sea ice climate models run under LGM and present-day conditions tend to underestimate the range of glacial-interglacial temperature changes in central Antarctica (Masson-Delmotte et al., 2006[29]): when considering an unchanged ice sheet elevation, the median simulated glacial-interglacial central Antarctic surface temperature change is 4.5°C. Future climate change in Antarctica, in response to anthropogenic CO2 emissions, is expected to reach 2°C in 100 years, which is much faster than the fastest change of the last transition (Masson-Delmotte et al., 2006[29]). Other IPCC models described later in this volume predict temperature changes of up to 4-6°C
Over the last transition in Antarctica, warming seems to start first, followed after a few hundred years by an increase in atmospheric CO2 concentrations (Monnin et al., 2001[11]). The rise in CH4 also appears to lag the initial rise in Antarctic temperature, but while the significant rapid excursions of CH4 apparent in the Greenland record (which are related to the abrupt warming into the Bølling-Allerød, the cooling to the Younger Dryas and the warming to the pre-Boreal) are mirrored in the Antarctic CH4 record, these rapid changes seem to have no direct climate parallel in Antarctic temperature. The abrupt Bølling-Allerød warming recorded in the Northern Hemisphere takes place at the beginning of the Antarctic Cold Reversal, but the precise cross-dating of these events remains disputed (Morgan et al., 2002[7]). Finally, the end of the Younger Dryas occurs when Antarctic temperatures have already reached their early Holocene optimum. The north-south sequence of events is affected by changes in orbital forcing, oceanic and atmospheric circulations, including see-saw effects related to changes in the thermohaline circulation, and biogeochemical cycle feedbacks. The precise role of Northern Hemisphere or Southern Hemisphere insolation changes on the termination onset remains uncertain (Schulz and Zeebe, 2006[30]). Similarly, and despite the strong correlation between CO2 variations and Antarctic temperature, the mechanisms involved in glacial-interglacial changes in greenhouse gas concentrations and their lags with Antarctic temperature are still a challenge for modellers (Köhler et al., 2005[31]).
Across the transition, the concentration of the majority of aerosol species falls from high glacial levels to lower levels in the Holocene. However, the concentration measured in ice is a combination of changes in both the atmospheric aerosol loading and the accumulation rate. While the use of concentration or flux (expressed in µg m-2 year-1) is still debated for high accumulation sites, it is generally agreed that for the low accumulation central Antarctic sites, where dry deposition dominates wet deposition, the ice core flux measurement gives a better indication of the initial atmospheric aerosol concentration (Fischer et al., 2007b). At Dome C, the flux of sea-salt sodium and non-sea-salt calcium decrease by factors of 2 and 30, while in Dronning Maud Land, they decrease by factors of 2.6 and 10. The coherence of the sea-salt records from both sites may indicate the waning of the sea ice cover in the Indian and Atlantic Ocean sectors, with the greater magnitude of changes in Dronning Maud Land attributed to a greater retreat in sea ice cover and an additional role of summer sea-ice extent in the Weddell Sea sector. The non-sea-salt Ca is the fraction that is considered representative of mineral dust of continental origin, and the likely source region for both Dronning Maud Land and Dome C is Patagonia (Smith et al., 2003[32]). The higher concentration of dust in Dronning Maud Land is indicative of a greater input of aeolian dust to the Weddell Sea region (Fischer et al., 2007a), presumably as a consequence of the closer proximity to the source region. Models have indicated that there is surprisingly little change in transport strength and atmospheric residence time of atmospherically entrained dust. Change in residence time makes no sense in this case between South America and Dome C during glacial and interglacial periods, so the large reduction in flux during the transition seen at both sites, which began at about 18 ka BP, is thought to be primarily resulting from the changes in the aridity and extent of the dust source region (Wolff et al., 2006[13]; Fischer et al., 2007a). An alternative hypothesis is that changes in the dust flux may be influenced by changes in the position of the polar front and strength as demonstrated by calibration of non sea-salt Ca and westerlies (Yan et al., 2005[33]).
Although the broad features of the last termination are now well documented in Antarctica, improvements can still be made in the detailed sequence of events with better resolution both temporally (for instance, in the phasing of changes in Antarctic climate, environmental parameters and atmospheric composition) and spatially, particularly in near coastal regions. This calls for further high resolution records of the last deglaciation and a comparison of records from ice drilling sites reflecting different oceanic basins. In addition, for a better understanding of climate dynamics, new ice cores are also needed to improve the knowledge of Antarctic ice sheet dynamics over the past deglaciation. Recently drilled records at Berkner Island and Talos Dome spanning the last deglaciation and beyond, and future new deep ice cores (Figure 3.15) will help to progress the understanding of the climate and biogeochemical cycle responses to climate forcings, the climate variability around Antarctica and its source areas, and the reaction of the Antarctic ice sheet to major climate changes of the past.
References
- ↑ 1.0 1.1 1.2 1.3 1.4 Rasmussen, S.O., Andersen, K.K., Svensson, A.M., Steffensen, J.P., Vinther, B.M., Clausen, H.B., Siggaard-Andersen, M.-L., Johnsen, S.J., Larsen, L.B., Dahl-Jensen, D., Bigler, M., Röthlisberger, R., Fischer, H., Goto-Azuma, K., Hansson, M.E. and, Ruth, U. 2006. A new Greenland ice core chronology for the last glacial termination, Journal of Geophysical Research, 111 , D06102.
- ↑ Spahni, R., Chappellaz, J., Stocker, T.F., Loulergue, L., Hausammann, G., Kawamura, K., Fluckiger, J., Schwander, J., Raynaud, D., Masson-Delmotte, V. and Jouzel, J. 2005. Atmospheric Methane and Nitrous Oxide of the Late Pleistocene from Antarctic Ice Cores, Science, 310, 1317-1321.
- ↑ 3.0 3.1 Jouzel, J., Masson-Delmotte, V., Cattani, O., Dreyfus, G., Falourd, S., Hoffmann, G., Minster, B., Nouet, J., Barnola, J.M., Chappellaz, J., Fischer, H., Gallet, J.C., Johnsen, S., Leuenberger, M., Loulergue, L., Luethi, D., Oerter, H., Parrenin, F., Raisbeck, G., Raynaud, D., Schilt, A., Schwander, J., Selmo, E., Souchez, R., Spahni, R., Stauffer, B., Steffensen, J.P., Stenni, B.S., Tison, J.L., Werner, M. and Wolff, E. 2007. Orbital and millenial Antarctic climate variability over the past 800,000 years, Science, 317, 793-796.
- ↑ 4.0 4.1 4.2 4.3 EPICA community members. 2006. One-to-one coupling of glacial climate variability in Greenland and Antarctica, Nature, 444, 195-198.
- ↑ Severi, M., Becagli, S., Castellano, E., Morganti, A., Traversi, R., Udisti, R., Ruth, U., Fischer, H., Huybrechts, P., Wolff, E., Parrenin, F., Kaufmann, P., Lambert, F. and Steffensen, J.P. 2007. Synchronisation of the EDML and EDC ice cores for the last 52 kyr by volcanic signature matching, Climate of the Past, 3, 367-374.
- ↑ 6.0 6.1 Blunier, T., Schwander, J., Stauffer, B., Stocker, T., Dallebach, A., Indermühle, A., Tschumi, J., Chappellaz, J., Raynaud, D. and Barnola, J.M. 1997. Timing of the Antarctic Cold Revearsal and the atmospheric CO2 increase with respect to the Younger Dryas event, Geophys. Res. Letters, 24, 2683 - 2686.
- ↑ 7.0 7.1 7.2 Morgan, V., Delmotte, M., Van Ommen, T., Jouzel, J., Chappellaz, J., Woon, S., Masson-Delmotte, V. and Raynaud, D. 2002. Relative Timing of Deglacial Climate Events in Antarctica and Greenland, Science, 297, 1862-1864.
- ↑ Berger, A. and Loutre, M.F. 1991. Insolation values for the climate of the last 10 million years, Quaternary Sciences Reviews, 10, 297-317.
- ↑ Lambeck, K. and Chappell, J. 2001. Sea level change through the last glacial cycle, Science, 292, 679-686.
- ↑ Waelbroeck, C., Labeyrie, L., Michel, E., Duplessy, J.C., McManus, J.F., Lambeck, K., Balbon, E. and Labracherie, M. 2002. Sea level and deep water temperature changes derived from bentic foraminifera isotopic records, Quat. Sci. Rev., 21, 295-305.
- ↑ 11.0 11.1 Monnin, E., Indermuhle, A., Dallenbach, A., Fluckiger, J., Stauffer, B., Stocker, T.F., Raynaud, D. and Barnola, J-M. 2001. Atmospheric CO2 Concentrations over the Last Glacial Termination, Science, 291, 112-114.
- ↑ Petit, J.R., Basile, I., Leruyuet, A., Raynaud, D., Lorius, C., Jouzel, J., Stievenard, M., Lipenkov, V.Y., Barkov, N.I., Kudryashov, B.B., Davis, M., Saltzman, E. and Kotlyakov, V. 1997. Four climatic cycles in Vostok ice core, Nature, 387, 359.
- ↑ 13.0 13.1 13.2 Wolff, E.W., Fischer, H., Fundel, F., Ruth, U., Twarloh, B., Littot, G.C., Mulvaney, R., Röthlisberger, R., De Angelis, M., Boutron, C.F., Hansson, M., Jonsell, U., Hutterli, M.A,, Lambert, F., Kaufmann, P., Stauffer, B., Stocker, T.F., Steffensen, J.P., Bigler, M., Siggaard-Andersen, M.L., Udisti, R., Becagli, S., Castellano, E., Severi, M., Wagenbach, D., Barbante, C., Gabrielli, P. and Gaspari, V. 2006. Southern Ocean sea-ice extent, productivity and iron flux over the past eight glacial cycles, Nature, 440, 491-496 (doi:10.1038/nature04614).
- ↑ Hammer, C.U., Clausen, H.B. and Langway, C.C.J. 1994. Electrical conductivity method (ECM) stratigraphic dating of the Byrd Station ice core, Antarctica, Annals of Glaciology, 20, 115-120.
- ↑ Yao, T., Petit, J.R., Jouzel, J., Lorius, C. and Duval, P. 1990. Climatic record from an ice margin area in East Antarctica, Annals of Glaciology, 14, 323-327.
- ↑ Petit, J.R., Jouzel, J., Raynaud, D., Barkov, N.I., Barnola, J.M., Basile, I., Bender, M., Chappellaz, J., Davis, J., Delaygue, G., Delmotte, M., Kotyakov, V.M., Legrand, M., Lipenkov, V.Y., Lorius, C., Pépin, L., Ritz, C., Saltzman, E. and Stievenard, M. 1999. Climate and Atmospheric History of the Past 420000 years from the Vostok Ice Core, Antarctica, Nature, 399, 429-436.
- ↑ Nikolaiev, V.I., Kotlyakov, V.M. and Smirnov, K.E. 1988. Isotopic studies of the ice core from the Komsomolskaia station, Antarctica. Data of Glaciological Studies of the USSR Academy of Sciences, 63, 97-102.
- ↑ 18.0 18.1 Jouzel, J., Vaikmae, R., Petit, J.R., Martin, M., Duclos, Y., Stiévenard, M., Lorius, C., Toots, M., Burckle, L.H., Barkov, N.I., Kotlyakov, V.M. 1995. The two-step shape and timing of the last deglaciation in Antarctica, Climate Dynamics, 11, 151-161.
- ↑ Steig, E.J., Brook, E.J., White, J.W.C., Sucher, C.M., Bender, M.L., Lehman, S.J., Morse, D.L., Waddington, E.D. and Clow, G.D. 1998. Synchronous climate changes in Antarctica and the North Atlantic, Science, 282, 92-95.
- ↑ Lorius, C., Merlivat, L., Jouzel, J. and Pourchet, M. 1979. A 30,000-yr isotope climatic record from Antarctic ice, Nature, 280, 644-648.
- ↑ EPICA community members. 2004. Eight glacial cycles from an Antarctic ice core, Nature, 429, 623-628.
- ↑ 22.0 22.1 Watanabe, O., Jouzel, J., Johnsen, S., Parrenin, F., Shoji, H. and Yoshida, N. 2003. Homogeneous climate variability across East Antarctica over the past three glacial cycles, Nature, 422, 509-512.
- ↑ Brook, E.J., White, J.W.C., Schilla, A.S.M., Bender, M.L., Barnett, B., Severinghaus, J.P., Taylor, K.C., Alley, R.B. and Steig, E.J. 2005. Timing of millenial-scale climate change at Siple Dome, West Antarctica, during the last glacial period, Quarternary Science Reviews, 24, 1333-1343.
- ↑ Masson-Delmotte, V., Hou, S., Ekaykin, A., Jouzel, J., Aristarain, A., Bernardo, R.T., Bromwhich, D., Cattani, O., Delmotte, M., Falourd, S., Frezzotti, M., Gallée, H., Genoni, L., Isaksson, E., Landais, A., Helsen, M., Hoffmann, G., Lopez, J., Morgan, V., Motoyama, H., Noone, D., Oerter, H., Petit, J.R., Royer, A., Uemura, R., Schmidt, G.A., Schlosser, E., Simões, J.C., Steig, E., Stenni, B., Stievenard, M., Broeke, M.V.D., Wal, R.V.D., Berg, W-J.V.D., Vimeux, F. and White, J.W.C. 2008. A review of Antarctic surface snow isotopic composition : observations, atmospheric circulation and isotopic modelling, J. Climate, 21, 3359-3387.
- ↑ Jouzel, J., Vimeux, F., Caillon, N., Delaygue, G., Hoffmann, G., Masson-Delmotte, V. and Parrenin, F. 2003. Magnitude of the Isotope/Temperature scaling for interpretation of central Antarctic ice cores, J. Geophys. Res., 108,1029-1046.
- ↑ Werner, M., Heimann, M. and Hoffmann, G. 2001. Isotopic composition and origin of polar precipitation in present and glacial climate simulations, Tellus, 53B, 53-71.
- ↑ 27.0 27.1 Stenni, B., Masson-Delmotte, V., Johnsen, S., Jouzel, J., Longinelli, A., Monnin, E., Rothlisberger, R. and Selmo, E. 2001. An Oceanic Cold Reversal During the Last Deglaciation, Science, 293, 2074-2077.
- ↑ Vimeux, F., Cuffey, K. and Jouzel, J. 2002. New insights into Southern Hemisphere temperature changes from Vostok ice cores using deuterium excess correction over the last 420, 000 years, Earth Planet. Sci. Lett., 2O3, 829-843.
- ↑ 29.0 29.1 29.2 Masson-Delmotte, V., Kageyama, M., Braconnot, P., Charbit, S., Krinner, G., Ritz, C., Guilyardi, E., Jouzel, J., Abe-Ouchi, A., Crucifix, M., Gladstone, R.M., Hewitt, C.D., Kitoh, A., Legrande, A., Marti, O., Merkel, U., Motoi, T., Ohgaito, R., Otto-Bliesner, B., Peltier, W.R., Ross, I., Valdes, P.J., Vettoretti, G., Weber, S.L. and Wolk, F. 2006. Past and future polar amplification of climate change: climate model intercomparisons and ice-core constraints, Climate Dynamics, 26, 513-529
- ↑ Schulz, K.G. and Zeebe, R.E. 2006. Pleistocene glacial terminations triggered by synchronous changes in Southern and Northern Hemisphere insolation : the insolation canon hypothesis, Earth and Planetary Science Letters, 249, 326-336.
- ↑ Köhler, P., Fischer, H., Munhoven, G. and Zeebe, R.E. 2005. Quantitative interpretation of atmospheric carbon records over the last glacial termination, Glob. Biogeochem. Cycles, 19, Art. No. GB4020.
- ↑ Smith, J., Vance, D., Kempa, R.A., Archer, C., Tomsa, P., Kinga, M. and Zárate, M. 2003. Isotopic constraints on the source of Argentinian loess-with implications for atmospheric circulation and the provenance of Antarctic dust during recent glacial maxima, Earth and Planetary Science Letters, 212, 181-196.
- ↑ Yan, Y., Mayewski, P.A., Kang, S. and Meyerson, E. 2005. An ice core proxy for Antarctic circumpolar wind intensity, Annals of Glaciology, 41, 121-130.