The ice sheet in the instrumental period
- This page is part of the topic Antarctic climate and environment change in the instrumental period
Contents
Introduction
Ice sheets are to some degree self-regulating systems. Increasing snowfall over the ice sheet will act to increase the ice thickness, but that will then increase the rate of ice-flow towards the coasts and thus remove the extra snowfall. Thus the ice sheet will evolve towards a shape and pattern of flow specific to the current climate, where flow exactly compensates the spatial pattern of ice accumulation (snowfall and frost deposition) and ice ablation (melting, wind erosion and calving).
This equilibrium state is a useful concept, but it is rarely realised; the driving environmental parameters of climate are themselves constantly changing, which continually modifies the equilibrium state sought by the ice sheet. At any given time, the changes in the ice sheet reflect responses to both recent and long-term changes in climate, and for this reason, areal extent, magnitude and duration of changes, as well as time scale must be carefully considered in any discussion of ice-sheet change.
Until the era of satellites, large-scale changes in the ice sheet were considered likely to unfold over thousands of years (Payne et al., 2006[1]). Now, significant accelerations and decelerations of large outlet glaciers and ice streams have been observed on much shorter timescales (Bindschadler et al., 2003[2]; Rignot and Kanagaratnam, 2006[3]; Truffer and Fahnestock, 2007[4]). In Antarctica, these changes affect a complex drainage system of ice streams and tributaries whose full extent has only become appreciated through satellite observations (Joughin et al., 1999[5]; Bamber et al., 2000[6]). Since the 1970s, there have been competing hypotheses concerning the influence of ice shelves on the flow rates of upstream glaciers. Satellites have confronted these hypotheses with empirical measurements for the first time. Another important contribution of satellites has been to identify two key regions of change in Antarctica; one near the northern tip of the Antarctic Peninsula, another within the rarely visited Amundsen Sea sector of West Antarctica.
Despite a good understanding of the complexity of the issues, its importance to understanding sea level rise has meant that measurement of the ice sheet’s mass balance has been a primary goal of Antarctic science since the early efforts following the IGY (1957/58). Many of these were based on accounting methods involving calculations of the imbalance between net snow accumulation and outflow of ice over particular domains. Such efforts have always been hampered by the intrinsic uncertainty in measuring these parameters, and very few have produced measurements of ice-sheet imbalance that do not plausibly allow changes in a particular domain to be either positive or negative. So while there have been a few notable exceptions (e.g. Joughin and Tulaczyk, 2002[7]; Rignot and Thomas, 2002[8]; Rignot, 2008[9]), and future efforts based on satellite data may prove to be valuable, our best measurements of change across the majority of the Antarctic ice sheet come not from accounting methods, but rather from those techniques that seek to measure the changing volume of the ice-sheet directly.
The most successful of these techniques of direct measurement has been the use of satellite altimetry (Figure 4.34). A number of research groups have evaluated data beginning in the early 1980s. Spanning data from multiple satellite altimeters, they have produced broadly consistent results (e.g., Wingham et al., 1998[10], 2006a[11]; Davis et al., 2005[12]; Zwally et al., 2005[13]). These results illustrate that separate catchment basins within the ice sheet behave somewhat independently. What altimetry often fails to capture are the largest changes at the ice sheet margins, where steep slopes lead to large errors of measurement, and the large thickness changes on the floating ice shelves at the perimeter, where elevation changes represent only 1/8 of the full thickness change.
More recently, measurements of changes in the Earth’s gravity field have been made using GRACE (Gravity Recovery and Climate Experiment). These satellites have confirmed that the Amundsen Sea sector is losing mass to the ocean (Velicogna and Wahr, 2006[14]; Ramillien et al., 2006[15]; Chen et al., 2006[16]). The GRACE system works by tracking the range between two orbiting satellites: their differential accelerations provide a sensitive measure of how the distribution of mass across the Earth’s surface is changing. The system is unable to distinguish separately the changes in ice, rock, and air; so the flows within the Earth’s mantle and atmosphere must be removed to reveal changes in the ice sheets. So far this correction has been derived using models of the atmospheric and lithospheric mass flows, but observations of isostatic uplift measured in the field using GPS receivers mounted on exposed rock outcrops should provide a better constraint, especially if GRACE observations are combined with altimetric observations (Velicogna and Wahr, 2002[17]). Chen et al. (2006[16]) report a localised region of mass increase in East Antarctica. This could either be anomalously high snowfall, leading to growth of ice in this region, or an artefact of un-modelled post-glacial rebound. The system has only been in operation for a few years, and snowfall is variable from year to year, so the long-term significance of the available results can be questioned. However there is little doubt that longer records from these satellites will eventually provide extremely valuable information on the changes in the mass of ice sheets.
Antarctica’s ice shelves and ice tongues (‘ice tongues’ are narrowly-confined ice shelves bounded by fjord walls) are particularly sensitive to climate change because both the upper and lower surface of the ice plate are exposed to different systems, each with a potential to cause rapid change: these are the atmosphere and the ocean. The ice-atmosphere system affects the ice shelf through changing surface accumulation, dust and soot deposition, and surface melt; the ice-ocean system controls basal melting or freezing, tidal flexure, and wave action. Moreover, it is now recognized that sea ice, which is not part of the ice sheet, but is viewed more properly as a component of the atmosphere-ocean system, has a large impact on the local climate and dynamics of the ice shelf front, controlling regional surface energy balance, moisture flux, and the presence or absence of ocean swell at the front.
Antarctica’s ice shelves have provided the most dramatic evidence to date that at least some regions of the Antarctic are warming significantly, and have shown, what has been suspected for long time (e.g. Mercer, 1978[18]), namely that changes in floating ice shelves can cause significant changes in the grounded ice sheet. This evidence has led to an ongoing re-assessment of how quickly greenhouse-driven climate changes could translate into sea level rise (Meehl et al, 2007[19]).
Ice shelves in two regions of the Antarctic Ice Sheet have shown rapid changes in recent decades: the Antarctic Peninsula and the northern region of West Antarctica draining into the Amundsen Sea. Because there is such spatial variety in the observed changes, and because the causes of the changes likely vary regionally, changes over the past 50 years are discussed below according to province. A review of events in these two regions will introduce two main mechanisms by which climate change is thought to lead to changes in ice sheet mass balance: surface summer melt increase, leading to ponding, fracturing and disintegration: and basal melting of the ice shelves, leading to shelf thinning and grounding line retreat.
Calving
Aside from the catastrophic ice shelf disintegration events discussed below, available data suggest that major rift-driven calving events have neither increased nor decreased on the major ice shelves (the Ross, Flichner-Ronne, or Amery). Rather there is ample evidence that their calving patterns continue to follow quasi-repetitive patterns extending back to the Nineteenth Century, when the ice fronts were first mapped (e.g. Jacobs et al., 1986[20]; Keys et al, 1990[21]; Lazzara et al., 1999[22]; Budd, 1966[23]; Fricker et al., 2005[24]; see also Frezzotti and Polizzi, 2002[25], and Kim et al., 2007[26]). So while the periodic calving of massive icebergs that appear to represent, in some cases, many decades of ice shelf advance, may appear dramatic, there is no reason to believe that they are not part of the normal fluctuations in a ice sheet that is, in the long-term, close to equilibrium.
Sub-glacial Water Movement
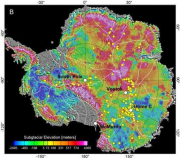
Regional surface elevation changes confined to areas of a few kilometres have been interpreted as manifestations of subglacial water movements (Gray et al., 2005[27]; Wingham et al., 2006b[28]; Fricker et al., 2007[29]). These observations are interpreted to reflect the activity of a subglacial hydrologic system permitting faster ice flow that is more active than previously thought (Figure 4.42). While those earlier studies lacked simultaneous measurements of ice flow to accompany these likely shifts in water mass, a recent study of Byrd Glacier identified a period of 10% faster flow that fell within a period where the nearby subglacial lakes discharged water that probably exited the system by traveling underneath Byrd Glacier (Stearns et al., 2008[30]). Improvements to numerical ice flow models are including subglacial water, but the specific nature of its role in ice sheet dynamics remains undetermined.
Effects of sedimentation on ice sheet stability
Much attention is focused on accelerating changes and instability, yet sediment deposited beneath fast moving outlet glaciers might provide some stability to ice sheet retreat driven by rising sea level. Recent observations and modeling suggest that wedges of sediment deposited near the grounding line may be important in stabilizing the ice sheet against sea level rise (Anandakrishnan et al. 2007[31]; Alley et al, 2007[32]). This stabilization is conditional on the position of the grounding line with respect to the crest of the wedge: should the grounding line retreat from the sediment wedge an unstable retreat analogous to that seen in tidewater glaciers could occur (Weertman, 1974[33]; Schoof, 2007[34]). It is becoming clear that rates of erosion and sediment transport can be large, as shown by the appearance of drumlin-scale sedimentary features underneath the ice sheet within just a few years (Smith et al., 2007a[35]). Predictive models of the ice sheet will need to include sediment transport, as well as forces imposed by ice shelves, since these effects may compete to determine the stability or instability of the ice sheet margin.
Pages in this topic
- The Antarctic Peninsula cryosphere in the instrumental period
- The West Antarctic cryosphere in the instrumental period
- The East Antarctic cryosphere in the instrumental period
- Attribution of ice sheet changes in the instrumental period
- Conclusions on the ice sheet in the instrumental period
References
- ↑ Payne, A.J., Sammonds, P. and Hunt, J.C.R. 2006, Evolution of the Antarctic ice sheet: new understanding and new challenges, Philosophical Transactions of the Royal Society A, 364, 1844.
- ↑ Bindschadler, R. A., King, M.A., Alley, R.B., Anandakrishnan, S. and Padman, L. 2003, Tidally controlled stick-slip discharge of a West Antarctic ice stream, Science, 301, 1087-1089.
- ↑ Rignot, E. and Kanagaratnam, P. 2006. Changes in the Velocity Structure of the Greenland Ice Sheet, Science, 311, no. 5763, 986-990.
- ↑ Truffer M. and Fahnestock, M. 2007. Rethinking Ice Sheet Time Scales, Science, 315, 1508.
- ↑ Joughin, I., Gray, L., Bindschadler, R., Price, S., Morse, D., Hulbe, C., Mattar, K. and Werner, C. 1999. Tributaries of West Antarctic ice streams revealed by Radarsat interferometry, Science, 286, 283-286, (doi:10.1126/science.286.5438.283).
- ↑ Bamber, J., Vaughan, D.G. and Joughin, I. 2000. Widespread complex flow in the interior of the Antarctic ice sheet, Science, 287, 1248-1250, (doi:10.1126/science.287.5456.1248).
- ↑ Joughin, I. and Tulaczyk, S. 2002. Positive mass balance of the Ross Ice Streams, West Antarctica, Science, 295 (Jan 18), No. 5554, 476-480.
- ↑ Rignot, E. and Thomas, R.H. 2002. Mass balance of polar ice sheets, Science, 297 (5586), 1502-1506 AUG 30 2002.
- ↑ Rignot, E. 2008. Changes in West Antarctic ice stream dynamics observed with ALOS PALSAR data, Geophysical Research Letters, 35, L12505, doi:10.1029/2008GL033365.
- ↑ Wingham, D.J., Ridout, A.L., Scharroo, R., Arthern, R.J. and Schum, C.K. 1998, Antarctic elevation change from 1990 to 1996, Science, 282, 456-458, (doi:10.1126/science.282.5388.456).
- ↑ Wingham, D.J., Shepherd, A., Muir, A. and Marshall, G.J. 2006a. Mass balance of the Antarctic ice sheet, Philosophical Transactions of the Royal Society A: Mathematical, Physical and Engineering Sciences, DOI: 10.1098/rsta.2006.1792.
- ↑ Davis, C.H., Li Y., McConnell, J.R., Frey, M.M. and Hanna, E. 2005. Snowfall-driven growth in East Antarctic Ice Sheet mitigates recent sea-level rise, Science, 308, 1898-1901.
- ↑ Zwally, H.J., Giovinetto, M., Li, J., Cornejo, H., Beckley, M., Brenner, A., Saba, J. and Yi, D. 2005. Mass changes of the Greenland and Antarctic ice sheets and shelves and contributions to sea-level rise: 1992-2002, Journal of Glaciology, 51(175), 509-527.
- ↑ Velicogna, I. and Wahr, J. 2006, Measurements of Time-Variable Gravity Show Mass Loss in Antarctica, Science, 311, (5768), 1754 DOI:10.1126/science.1123785.
- ↑ Ramillien, G., Lombard, A., Cazenave, A., Ivins, E.R., Llubes, M., Remy, F. and Biancale, R. 2006. Interannual variations of the mass balance of the Antarctica and Greenland ice sheets from GRACE, Global and Planetary Change, 53, 198-208.
- ↑ 16.0 16.1 Chen, J. L., Wilson, C.R., Blankenship, D.D. and Tapley, B.D. 2006. Antarctic mass rates from GRACE, Geophys. Res. Lett., 33, L11502, doi:10.1029/2006GL026369.
- ↑ Velicogna, I. and Wahr, J. 2002. A method for separating Antarctic postglacial rebound and ice mass balance using future ICESat Geoscience Laser Altimeter System, Gravity Recovery and Climate Experiment, and GPS satellite data, J. Geophys. Res., 107, 10.1029/2001JB000708.
- ↑ Mercer, J.H. 1978. West Antarctic ice sheet and CO2 greenhouse effect: a threat of disaster, Nature, 271, 321-325.
- ↑ Meehl, G.A., T.F. Stocker, W.D. Collins, P. Friedlingstein, A.T. Gaye, J.M. Gregory, A. Kitoh, R. Knutti, J.M. Murphy, A. Noda, S.C.B. Raper, I.G. Watterson, A.J. Weaver, and Z.-C. Zhao, 2007: Global Climate Projections. In: Climate Change 2007: The Physical Science Basis. Contribution of Working Group I to the Fourth Assessment Report of the Intergovernmental Panel on Climate Change. Cambridge University Press, Cambridge, United Kingdom and New York, NY, USA, 749-845.
- ↑ Jacobs, S.S., Macayeal, D.R. and Ardai, J.L., Jr., 1986. The recent advance of the Ross Ice Shelf, Antarctica, Journal of Glaciology, 32(112), 464-474.
- ↑ Keys, H., Jacobs, S. and Barnett, D. 1990. The calving and drift of iceberg B-9 in the Ross Sea, Antarctica, Antarctic Science, 2(3), 243-257.
- ↑ Lazzara, M.A., Jezek, K.C., Scambos, T.A., Macayeal, D.R. and Van Der Veen, C.J. 1999. On the recent calving of icebergs from the Ross Ice Shelf, Polar Geography, 23(3), 201-212.
- ↑ Budd, W. 1966. The dynamics of the Amery Ice Shelf, Journal of Glaciology, 6, 335-358.
- ↑ Fricker, H.A., Young, N.W., Coleman, R., Bassis, J.N. and Minster, J.-B. 2005. Multi-year monitoring of rift propagation on the Amery Ice Shelf, East Antarcitca, Geophysical Research Letters, 32, L02502, doi:10.1029/2004GL021036.
- ↑ Frezzotti, M. and Polizzi, M. 2002. 50 years of ice-front changes between the Adélie and Banzare Coasts, East Antarctica, Annals of Glaciology, 34, 235-240.
- ↑ Kim, K., Jezek, K.C. and Liu, H. 2007. Orthorectifield image mosaic of Antarctica from 1963 Argon satellite photography: image processing and glaciological applications, International Journal of Remote Sensing, 28(23), 5357-5373, doi: 10.1080/01431160601105850.
- ↑ Gray, L., Joughin, I., Tulazcyk, S., Spikes, V.B., Bindschadler, R. and Jezek, K. 2005. Evidence for subglacial water transport in the West Antarctica Ice Sheet through three-dimensional satellite radar interferometry, Geophysical Research Letters, 32, L03501, doi:10.1029/2004GL021387,.
- ↑ Wingham, D.J., Siegert, M.J., Shepherd, A. and Muir, A.S. 2006b. Rapid discharge connects Antarctic subglacial lakes, Nature, 440, pp. 1033-1036 , doi:10.1038/nature04660.
- ↑ Fricker, H.A., Scambos, T.A., Bindschadler, R. and Padman, L. 2007. An Active Subglacial Water System in West Antarctica Mapped from Space, Science, 315, 1544, doi: 10.1126/science.1136897.
- ↑ Stearns, L.A., Smith, B.E. and Hamilton, G.S. 2008. Increased flow speed on a large East Antarctic outlet glacier caused by subglacial floods, Nature Geoscience, 1, 827-831.
- ↑ Anandakrishnan, S., Catania, G.A., Alley, R.B. and Horgan, H.J. 2007. Discovery of till deposition at the grounding line of Whillans Ice Stream, Science, 315, 1835-1838.
- ↑ Alley, R.B., Anandakrishnan, S., Dupont, T.K., Parizek, B.R. and Pollard, D., 2007, Effect of sedimentation on ice-sheet grounding-line stability. Science, 315, 1838-1841.
- ↑ Weertman, J. 1974. Stability of the junction of an ice sheet and an ice shelf, J. Glaciol., 13, 3-11.
- ↑ Schoof, C. 2007. Marine ice sheet dynamics. Part I: The case of rapid sliding, J. Fluid. Mech., 573, 27-55.
- ↑ Smith, A.M., Murray, T., Nicholls, K.W., Makinson, K., Aðalgeirsdóttir, G., Behar, A.E. and Vaughan, D.G. 2007a. Rapid erosion, drumlin formation, and changing hydrology beneath an Antarctic ice stream, Geology, 35, 127-130.