Sea ice biological and physical modeling
- This page is part of the topic Models of the physical and biological environment of the Antarctic
Most of our knowledge on sea ice microalgal growth and production was derived from observational data and some experimental work. Recently, new experimental data have revealed the complex and heterogeneous character of the sea ice ecosystem (Thomas and Dieckmann, 2002[1]), and the new information is improving the parameterization of biological sea ice models . The dependence of microalgal growth on light, nutrient and temperature is mechanistic, which means all processes involved are in accordance with purely physical and chemical relationships. However it has often been modeled using empirical relationships, where microalgal growth is parameterized as a function of the most limiting resource (Liebig’s law). The difference between empirical and mechanistic models resides in the use of self-adapting variables by the latter to predict future states of microalgal biomass, allowing a dynamic adaptation of the biological processes to transient environmental changes. Such a dynamical approach depends on the physiological status of the cells. For Antarctic sea ice, where microalgal cells are subject to strong seasonal and daily variations in light, temperature and nutrients, only a mechanistic model can well represent the physiological responses of microalgal cells to environmental changes. The sea ice ecosystem differs considerably from water column conditions, and is characterized by extremely low temperatures, high brine salinities and strong downward attenuation of solar radiation. The sea ice seasonal cycle has two main implications for the spatial distribution of its microalgal communities: first, the ice growth season provides a large space surface area available for colonization in e.g. the brine channels of newly formed sea ice, followed by a drastic ice retreat during the transition to summer, dispersing the ice communities into the water column; and second, the role of ice drift during the growth season in the lateral transport of biomass through the seasonal ice zone, which significantly contributes to the horizontal distribution of food resources for other organisms associated with sea ice (e.g. the Antarctic krill and upward organisms further up in the trophic chain). The link between the establishment of microalgal communities and sea ice formation itself begins during direct interactions of ice crystals formed in seawater with individual organisms in the water column. This process is not selective and the mechanism by which microalgal cells, organic matter and other organisms are incorporated into developing sea ice is described in detail by Garrison et al. (1989[2]) and Weissenberger and Grossmann (1998[3]). Garrison (1991[4]) demonstrated that although the large number of species that inhabit the underlying water column and sea ice brine channels (bacteria, microalgae, protists, small metazoans and some crustaceans), the extreme environmental conditions which the organisms are exposed to are very selective, and most of the sea ice biomass is dominated by small diatoms which are responsible for almost all sea ice primary production. Gleitz et al. (1998) showed that for more than 100 different diatoms species already found in sea ice habitats, less than 20 contribute significantly to the total biomass commonly found in the ice-pack while Lizotte (2001[5]) inferred that Fragilartiopsis cylindrus and F. curta are the most dominant microalgae in sea ice. This low diversity may be related to the physiological capacity of these diatoms to maintain relatively high growth rates under extreme conditions of low light and temperature when compared to water column species. This apparent dominance of few species is also found between heterotrophic protists that inhabit sea ice. Garrison and Buck (1989[6]), working with pack-ice microbial communities in the Weddell Sea found that ciliates contributed 70% of the total protozoa biomass, followed by heterotrophic flagellates and other small protists, which play a key role in the cycling of material (e.g. excretion of nitrogen) and in controlling microalgal growth (Garrison, 1991[4]).
The first attempt to model the Antarctic sea ice primary production was made by Arrigo et al. (1993[7]), where the prognostic variables (C, N and Si) were modeled using static physiological parameterizations as a function of light, temperature and salinity, using a nutrient limitation scheme based on Monod growth kinetics and the Liebig’s law. The thermterm static comes from the absence of variables in the model that describe the state of the cells, as well as the use of Redfield ratios to couple the microalgal growth function with the uptake of N and Si to carbon. The Carbon-equivalent (Ceq) for the nutrients N and Si used by Arrigo and co-workers were C:N:Si of 107:17:40 molar-ratio, which was consistent with some sea ice observations (see details in Arrigo et al., 1995[8], 1997[9]). The shortcoming arising from such an approach is that microalgal growth limitation by light, temperature or salinity, affects only the rate of carbon incorporation in biomass, without any co-limitation and the system is basically controlled by the balance of nutrients and biomass in a fixed ratio (given by the molar ratios). When the environment is nutrient depleted, than growth rate relies only on the Monod growth kinetics. Thus, their model results show that the maximum biomass produced by microalgae depends only on the nutrient availability, and the rate of remineralization. The exact concentration is only related to the molar ratios between carbon, nitrogen and silicon. Although Redfield Ratios are commonly in use by many modelers (Arrigo et al., 1995[8]; Fritsen et al., 1998[10]), there are many cases where elemental composition does not match with these ratios and the use of Redfield is questionable.
There are many approaches to creating a biological model for sea ice microalgal growth and production and modern studies shown that a quasi-realistic model must be based on self-adapting mechanisms to relate the physiological status of sea ice microalgae to their primary production, including grazing by sea ice protozoa. Sea ice microalgae in models must grow over two essential co-limiting nutrients, dissolved inorganic nitrogen [N] and silicate [Si], and biomass is represented in terms of carbon content (P C), chlorophyll-a (PChl), particulate organic nitrogen (PN) and biogenic silica (PSi), allowing variable cellular N:C and Si:C quotas to decouple primary production and inorganic nutrient availability. Light- and temperature dependence of microalgal growth must be included in the model, treated by a set of ordinary differential equations, which describes the balance between light and the chlorophyll-a:carbon ratio, to simulate the photo acclimation mechanism. There is a lack of data about heterotrophic protists feeding rates on microalgae accumulating carbon and nitrogen biomass (ZC and ZN, respectively) and excreting the N-excess to the medium. However, remineralization of silicon is neglected in most experiments, as well as the uptake of other elements, like phosphate, iron, aluminum and vitamins.
Developing a sea ice coupled biological-physical model, to investigate the influence of transient changes in environmental conditions on sea ice biological communities, must include physiological self-adaptive schemes to simulate microalgal photoacclimation and uptake of dissolved nutrients in response to transient changes of light, temperature and nutrient supply. Grazing may be simulated by the incorporation of heterotrophic protists in the model with specific organic carbon and nitrogen pools, although there is almost no field data about these points. The excretion of nitrogen excess by the sea ice protozoa in some laboratory experiments proved to be of importance in sea ice nutrient dynamics.
Also, to simulate sea ice biology, the model must be coupled with a one-dimensional thermodynamic sea ice model including a numerical scheme to resolve the heat conduction in sea ice, as well as the incorporation and vertical redistribution of biological material due to brine flux. Belem (2002[11]) showed that these physical processes play a key role in the vertical structuring of sea ice biological communities and their associated primary production. The inclusion of brine fluxes in the physical model shows that vertical differentiation in the salinity profiles, commonly observed in ice cores collected in the Antarctic pack ice, results from variable ice growth rates and consequently salt partitioning, which cause distinct bulk salinity profiles. These differences associated with the vertical position of the impermeable layer play a key role in the formation of banded layers where high accumulation of biological material is commonly observed.
However, there are a few key questions to be assessed in such coupled biological-physical model. One of these is a Lagrangian approach, where the time-dependent position of simulated floes extracted from ice velocity fields can be used to compute model forcing parameters (e.g. air temperature, oceanic heat flux, solar radiation). These processes are essential to determine the thermodynamic history of floes and to obtain a good agreement between model results and field observations. During the drift, synoptical changes in environmental conditions lead to a differentiation of vertical sea ice characteristics that most models do not take into account. With exception of the model cited, all other sea ice models have an Eulerian approach.
One of the most important environmental parameters governing the sea ice primary production is the incoming solar radiation. The model includes the simulation of the spectral solar radiation attenuated by the atmosphere and clouds and a bio-optical sea ice model, determining the vertical distribution of light within sea ice. The solar radiation is also of significant importance for the sea ice thermodynamics, governing the temperature profile within sea ice during the summer months. Simulations with the spectral bio-optical sea ice model in many works showed that part of the marginal pack ice during the winter receives enough light to support biological production. This fact is of extreme importance for overwintering organisms associated with the sea ice.
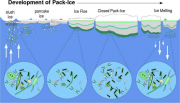
Thermodynamic processes controlling ice formation are fundamental in governing the vertical distribution of biological communities and the impermeable layer formed due to vertical temperature gradients and brine segregation acts as a barrier to the flux of biogenic material entrapped in the sea ice. Observed vertical variability in chlorophyll profiles from collected ice cores in the Weddell Sea are caused mainly by differential incorporation rates of biological material during the ice growth. Figure 2.38 represents the seasonal cycle of the Antarctic pack ice with emphasis on the physical processes controlling the incorporation and redistribution of biological material within sea ice.
During autumn, dynamic accumulation of microorganisms in the water column due to frazil ice scavenging results in high incorporation rates of biological material within newly accreted ice layers. During winter, the thermodynamic growth of the pack ice controls the vertical distribution of ice assemblages, mainly due to the effect of the brine flux. With the onset of the melting season in spring and summer and increasing solar radiation over sea ice, the biological activity reaches its maximum followed by a rapid decline in the primary production rates between February and March. The sea ice retreat constrains the primary productivity to persistent ice fields in the summer, characterized by nutrient depleted conditions and heavy snow cover, indicating a significant degree of limitation for microalgal production.
Belem (2002[11]) demonstrated in a coupled biological-physical sea ice model that the total sea ice productivity in the Weddell Sea shows an annual carbon production of approximately 11 Tg C. During the winter, the sea ice productivity ranges from 0.16 to 0.6 Tg C per month, contributing to 17% of the total annual production. These results are lower than previous estimates for the Weddell Sea pack ice computed only for infiltration communities associated with flooded snow and snow-ice formation. However, the coupled biological-physical sea ice models described before do not consider the formation of snow-ice and, therefore, infiltration communities are not taken into account. Snow flooding is restricted to the later spring/early summer in some areas of the Weddell Sea and cannot be generalized to the whole seasonal ice zone. Alternatively, most of the ice cores collected in the Weddell Sea indicate that infiltration communities occur in only a small fraction of the pack ice and model results revealed a dominance of interior and bottom ice assemblages.
The ecological distinction between these two assemblages is relevant to understanding the time course of sea ice colonization. Garrison and Buck (1991[12]) found that most of the sea ice infiltration assemblages are contained within a porous layer of hard ice near the freeboard level (i.e. the space between the ice surface and the seawater level) and apparently developed from internal assemblages. They also showed that interior assemblages are apparently established at the time of ice formation and thus overwinter survival in the ice is an important consideration. In contrast, an infiltration assemblage, which is inoculated when flooding occurs, would be heavily influenced by the composition of the plankton at the time of flooding. The results of coupled sea ice models suggest that the surface assemblages are related to the impermeable characteristic of topmost ice layers. However, the low temperatures associated with this vertical level must limit primary production.
Therefore, any estimates of the sea ice primary production obtained by models should be considered conservative, since the simulated pack ice consisted only of first-year ice. Many other ice habitats like multi-year ice, rafted ice floes, refrozen leads, infiltration layers, which are potential sites for the settlement of biological sea ice assemblages were not considered. The contribution of these communities to the total sea ice primary production needs to be further investigated. However, sea ice coupled physical and biological models are good tools to understand biological processes and its physical driven processes.
References
- ↑ Thomas, D.N. and Dieckmann, G.S. 2002. Antarctic sea ice-a habitat for extremophiles, Science, 295, 641-644.
- ↑ Garrison, D.L., Close, A.R. and Reimntz, A. 1989. Algae concentrated by frazil ice: evidence from laboratory and field measurements, Antarctic Sci., 1, 313-316.
- ↑ Weissenberger, J. and Grossmann, S. 1998. Experimental formation of sea ice: importance of water circulation and wave action for incorporation of phytoplankton and bacteria., Polar Biol., 20, 178-188.
- ↑ 4.0 4.1 Garrison, D.L. 1991. Antarctic sea ice biota, Am. Zool., 31, 17-33.
- ↑ Lizotte, M.P. 2001. The contributions of sea ice algae to. Antarctic marine primary production, Am. Zool., 41, 57-73.
- ↑ Garrison, D.L. and Buck, K.R. 1989. The biota of Antarctic pack ice in the Weddell sea and Antarctic peninsula regions, Polar Biol., 10, 211-219.
- ↑ Arrigo, K.R., Kremer, J.N. and Sullivan, C.W. 1993. A simulated Antarctic fast ice ecosystem, Journal of Geophysical Research, 98(C4), 6929-6946.
- ↑ 8.0 8.1 Arrigo, K.R., Dieckmann, G., Gosselin, M., Robinson, D.H., Fritsen, C.H. and Sullivan, C.W. 1995. High resolution study of the platelet ice ecosystem in McMurdo Sound, Antarctica: Biomass, nutrient, and production profiles within a dense microalgal bloom, Marine Ecology Progress Series, 127(1-3), 255-268.
- ↑ Arrigo, K.R., Worthen, D.L., Lizotte, M.P., Dixon, P. and Dieckmann, G. 1997. Primary production in Antarctic sea ice, Science, 276(5311), 394-397.
- ↑ Fritsen, C.H., Ackley, S.F., Kremer, J.N. and Sullivan, C.W. 1998. Flood-freeze cycles and microalgal dynamics in Antarctic pack ice. In: Lizotte MP, Arrigo K (eds) Antarctic sea ice: biological processes, interactions and variability, vol 73. American Geophysical Union, Washington, DC, 1-21.
- ↑ 11.0 11.1 11.2 Belem, A.L. 2002. Modeling physical and biological processes in Antarctic sea ice. Ph.D. thesis, Alfred Wegener Institute for Polar and Marine Research, Bremerhaven.
- ↑ Garrison, D.L. and Buck, K.R. 1991. Surface-layer sea ice assemblages in Antarctic pack ice during the austral spring: Environmental conditions, primary production and community structure, Marine Ecology Progress Series, 75, 161-172.