Meteorological and ozone observing in the Antarctic
- This page is part of the topic Observations, data accuracy and tools
Contents
Meteorological observations
The International Geophysical Year (IGY) provided a significant impetus towards setting up continuously operating stations in the Antarctic, and over forty were established, of which over a dozen are still operating today, alongside more recently established stations. This was the peak of manned observation in Antarctica, and since then the number of staffed stations has declined, though this is offset by an increasing number of automatic stations. A further boost to the observing network has taken place in the International Polar Year of 2007 – 2008.
Most manned stations are built on ice-free ground at coastal sites, primarily so that stores can easily be transported ashore and to simplify construction. The weather recorded at these sites is not a true representation of the continent as a whole, as the coastal areas are much milder than the interior due to the moderating influence of the sea and, at the more local scale, the surrounding rock. Automatic stations are much more widely spread across the continent and give a broader picture of the meteorology.
At most manned stations meteorological observations are made regularly throughout the “day” according to WMO standards, but there is increasing reliance on automatic systems during the “night”. Surface temperature, humidity, solar radiation, atmospheric pressure, wind speed and direction are largely measured by automated instruments, but an observer is needed to estimate visibility and the amount, type and height of clouds, although automatic instruments are being introduced to deal with these parameters. The observer also needs to monitor the weather: rain, snow, fog, gales etc., as well as noting more unusual phenomena: diamond dust, halos, mirages and the aurora australis. Traditional weather observing on the polar plateau brings additional problems, with the combination of very low temperature and high altitude.
The observations are expressed in a numeric code and sent via geostationary satellites to meteorological centres, largely in the Northern Hemisphere, where they join thousands of other observations from all over the world. They are processed by super-computers and used to forecast the weather.
Automatic weather stations (AWS; Figure 2.1 and Figure 2.2) generally measure a reduced range of parameters compared to the staffed stations, usually just atmospheric pressure, temperature and wind, although some may measure humidity, along with snow depth, which can also be used to estimate when the instruments may become buried. Where there is significant snow accumulation these stations require annual maintenance visits, however others may not be revisited after deployment.
Stations near the Antarctic coast are quite cloudy because of the frequent passage of depressions and the influence of the sea. The further a station is inland, the less cloudy it becomes. Signy has an average cloud cover of 86%, Halley 66% and the South Pole an average of 41%. Visual observation of cloud height is difficult at stations on ice shelves or the polar plateau, where the high albedo reduces contrast and there are no references to estimate height. Cloud lidars improve these measurements, and can also monitor precipitation falling from clouds.
The Antarctic atmosphere is very clear, as there are few sources of pollution. On a clear day it is possible to see mountains well over 100 km away. In these conditions, estimating distances can be very deceptive. Objects may appear to be close by, when in fact it would take many hours of travel to reach them. Automatic instruments, which use infra-red scintillation and scattering to measure visibility are becoming more common, although some have difficulty in discriminating variation in visibility above 20 km horizontal distance.
At approximately a dozen stations balloons are launched once or twice a day, each carrying a package of meteorological instruments known as a radiosonde. The instrument package signals back to base the temperature, humidity and pressure up to an altitude of over 20 km, with wind speed and direction being found by tracking the package with GPS sensors. One particular problem affects some balloons during winter: the combination of low ambient temperature and lack of heating from solar radiation makes the balloon fabric brittle and they burst early. The traditional remedy is to briefly dip the balloon in a mixture of oil and avtur immediately prior to launch, and to allow excess fluid to drain off. This plasticizes the fabric and gives much improved performance.
Special balloon ascents are sometimes made to help study the lower part of the atmosphere called the troposphere, where weather systems are active. These include flights to investigate very stable conditions in the lowest layer, which mainly occur during the winter, and other flights to study, for example, depressions forming off shore. Such studies are augmented by atmospheric profiles measured using captive packages carried aloft by kites or blimps, or by sodars (sonic radars). Further studies are made using instrumented aircraft, for example to study the composition of clouds in situ.
Ozone
Ozone is a compound of oxygen containing three atoms instead of the two in the oxygen we breathe. The largest concentrations of ozone in the atmosphere are found above the well-mixed lower atmosphere (the troposphere). The sunlight absorbed by these large concentrations of ozone heats the atmosphere locally, so the temperature increases with height, which leads to very little vertical wind in this ozone layer – hence its other name, the stratosphere. Above Europe, the ozone layer is typically from 13 to 50 km altitude. In Antarctica it is a few kilometres lower in altitude because of the reduced convective mixing of the troposphere in the reduced sunlight there, and because the colder temperatures result in higher densities that cause the same pressures to be at lower altitudes.
Ozone is created by the photochemical destruction of oxygen, which liberates a free oxygen atom to combine with an oxygen molecule:
- O2 + hν → 2O where hν is a photon of radiation of wavelength < 240 nm
- O2 + O + M → O3 + M where a third molecule (usually nitrogen or oxygen), M, is required to sequester or remove excess energy
The total amount of ozone in the atmosphere is routinely measured by a Dobson spectrometer, which measures the ratio of intensities of sunlight at two ultraviolet wavelengths between 305 and 340 nm, one of which is absorbed strongly by ozone, the other weakly. Wavelengths are selected by the use of prisms and a series of slits. A well-calibrated instrument can measure the amount of ozone to within a few percent. A typical amount of ozone in the atmosphere is around 300 milli-atmosphere-centimetres, equal to 300 Dobson Units (DU). If this was pure ozone at sea level, it would form a layer in the atmosphere just 3 mm thick.
Vertical profiles of ozone are measured by electrochemical sondes on balloons. Air is pumped through a cell containing potassium iodide solution, which generates a current proportional to ozone concentration. Ozone concentration is usually low in the troposphere, but increases in the stratosphere to a maximum between 15 and 25 km, above which ozone concentration decreases.
In common with most Antarctic data sets, that of total ozone in Antarctica starts in 1956 when stations began operation in the run up to the IGY of 1957 to 1958. Later some stations began measuring the vertical profiles of ozone by sondes. In the 1970s ozone measurements from polar orbiting satellites began. The long record of total ozone from Halley (Figure 2.3) and its location within the winter polar vortex made it the best site for discovering what is now known as the Antarctic ozone hole (Farman et al., 1985[1]).
The ozone hole was discovered via ground-based observations from Antarctica, and most manned stations continue with long-term measurements of the ozone column from the ground. Ground based sensors include the traditional Dobson ozone spectrophotometer, the Brewer spectrometer and the SAOZ (Système d'Analyse par Observation Zénithales) spectrometer, or variants of these. All measure the differential absorption of sunlight as it passes through the ozone layer. At a few stations ozone sondes are flown on balloons; these sondes give precise profiles of the ozone in the atmosphere.
Observational problems
Although many of the meteorological observations made in Antarctica are done in exactly the same way throughout the world, some additional problems are encountered. Temperature measurements are often made using a platinum resistance or a traditional thermometer in a Stephenson screen, or variations of it. The screen shields the thermometer from direct solar radiation and from precipitation falling on the thermometer. In low wind speeds in summer, the radiation reflected from the high albedo surface can give anomalously high readings, whilst under clear skies in winter the reverse can occur. Many stations use aspirated screens, where air is sucked over the thermometer bulb at a constant flow rate, to provide more consistent data. A further problem occurs below -38°C, when mercury freezes, and so is either doped with thalium for use down to -61°C, or coloured ethanol is used as a fluid. In blizzard conditions a screen can fill with drifted snow, giving a uniform temperature environment unless it is quickly cleared. Some protection can be afforded by the use of snow boards, which temporarily block the louvres whilst the blizzard is in progress.
Measuring the precipitation itself can be difficult. The snow is generally dry and any that falls into a standard rain gauge just as easily blows out again. Equally, precipitation that has fallen elsewhere or at a previous time can be blown around by the wind and into the gauge. Specially designed snow gauges provide a partial solution, and another is to measure the depth of freshly fallen snow, and assume that in the long term there is a balance between transported and falling snow. Electronic precipitation detectors using scintillation in an infrared beam are now being deployed in Antarctica, and combination of the outputs of two detectors at different heights may provide the necessary discrimination between precipitation and transport.
Wind measurements were traditionally made using large, heavy cup anemometers. These required a significant wind speed before they started turning and did not respond well to gusts. They were replaced by anemometers with light-weight plastic cups, which performed better in these circumstances, but which frequently became coated with rime deposited from fog near the coastal regions, and hence under recorded wind speeds. Propeller type combined vanes and anemometers suffer less from rime, but can fail mechanically. The modern replacement is the sonic anemometer, which can measure both wind-speed and direction, can be heated to dispel any riming, and has no moving parts apart from the ultrasonic source. Even these suffer problems, particularly in conditions of heavy blowing snow, when the pinging of snow grains on the sensor saturates the detector, so they do not have widespread use over the continent as of this date.
Sunshine amounts were traditionally measured using the Campbell-Stokes recorder, a sphere of glass that focuses the sun’s rays onto a fixed card, where they burn a hole in bright sunshine. This suffers from a universal problem of over-recording in patchy cloud conditions, and also experiences another problem in polar latitudes, where there can be 24 hours of daylight. In high southern (or northern) latitudes two recorders have to be mounted back to back to measure sunshine throughout the long day. Modern electronic recorders get around both problems and allow continuous recording, and are often more effective at recording sunshine at low solar elevations.
Early measurements of humidity were made using dry and “wet” bulb psychrometry. For much of Antarctica the “wet” bulb is an ice bulb, and a thin ice layer needs to be maintained for the technique to work reliably. This is not practical in an automatic system, and most sensors now use a capacitive technique to measure humidity. These sensors are generally reliable, although suffer a decline in accuracy when exposed to frequent freeze/thaw cycles. These conditions are common at many coastal sites, so that annual replacement of sensors often becomes necessary.
Data archiving
The Antarctic in-situ meteorological observations have been brought together within a SCAR project called REference Antarctic Data for Environmental Research (READER). The primary sources of data are the Antarctic research stations and automatic weather stations, and the data have, where possible, been obtained from the operators who run the stations. The observations have been quality controlled and are available on the web. For more details see http://www.antarctica.ac.uk/met/READER/. For periods prior to the instrumental era of Antarctic meteorological stations, ice core records that have been calibrated to observed data can provide highly valuable proxies. Data for Antarctic ice cores has been assmbled together within a SCAR project called Ice REference Antarctic Data for Environmental Research (Ice READER) (Figure 2.4) available on the web. For more details see http://www.icereader.org/icereader/.
Meteorological analyses
Some surface synoptic weather charts were prepared in the early years of the Twentieth Century, but these did not have a great deal of accuracy in the Antarctic, as there were very few observations over ocean areas. From the 1970s, an increasing number of observations became available from the polar orbiting weather satellites, which allowed the production of increasingly reliable atmospheric analyses at high southern latitudes. Of particular importance are the atmospheric temperature sounders that have been flown on the polar orbiting satellites since the mid 1970s. These provide profiles of temperature and humidity from the surface into the stratosphere and are similar in nature to the radiosonde ascents, although with the broad coverage provided by a satellite system.
Over the last few years the historical archives of in-situ and satellite observations have been re-processed using current data assimilation schemes to produce the so called ‘re-analysis’ data sets, which provide a particularly valuable source of information for investigation of climate variability over approximately the last three decades. However, prior to satellite sounder data becoming available in 1974, the re-analysis fields for the Southern Hemisphere are poor and cannot be used to investigate atmospheric circulation change.
Satellite Sounding of the Atmosphere
Satellite sounding instruments measure radiation at infrared or microwave wavelengths that has been emitted by the atmosphere itself, and thus provide information on the temperature and composition (e.g. humidity, ozone amount) of the atmosphere over a range of altitudes. Such instruments have improved our knowledge of the climate of the polar regions, and hence the forecasts made by Numerical Weather Prediction (NWP) models. Today, regions such as the Southern Ocean, previously a data void, are filled by data with a spatial resolution of tens of kilometres. The wavelengths of these types of instrument are selected according to the absorption and emission properties of carbon dioxide, nitrous oxide, oxygen, ozone and water vapour. As the distribution of these gases is relatively constant and their radiative properties understood, variations in the radiance received by the satellite (the brightness temperature) can be related via mathematical inversion methods to the temperature of that part of the atmosphere from which most of the signal emanates. By using a number of wavelengths that have different absorption characteristics a complete profile of temperature through the atmospheric column can be derived (see Figure 2.5).
The principal satellite sounding instruments are the TIROS-N operational vertical sounder (TOVS) sensor systems, which have flown on polar-orbiting satellites since 1978, and their successor Advanced TOVS (ATOVS), which began operating in 1998. TOVS comprises three radiometer arrays: (i) the high-resolution infrared radiation sounder version 2 (HIRS/2), the microwave sounding unit (MSU), and the stratospheric sounding unit (SSU), while in ATOVS the Advanced Microwave Sounding Unit-A (AMSU-A) and the Advanced Microwave Sounding Unit-B (AMSU-B) replace the MSU and the SSU, and HIRS/3 replaces the HIRS/2. Significant improvement in forecast accuracy has come from using the AMSU data.
Unfortunately, comparison against radiosondes launched from Arctic sea ice demonstrated marked deficiencies in the original TOVS retrieval algorithms over sea ice during the winter (Francis, 1994[2]). Near-surface temperature inversions were missed, primarily because of surface temperature retrievals over sea ice being 5-15°C too high. This was traced to a tendency for the algorithm to over-estimate total column water vapour (Francis, 1994[2]). Similar problems have been identified in the ECMWF 40 year reanalysis (ERA-40) prior to 1996 (Bromwich et al., 2007[3]). In the Antarctic there are the additional problems that the high Antarctic Plateau causes for an un-tuned retrieval algorithm (Lachlan-Cope, 1992[4]). However, a comparison of integrated water vapour from AMSU-B and limb-sounding observations by a GPS receiver on board the Low Earth Orbiter (LEO) CHAMP satellite (obtained using radio occultation techniques) showed good agreement between the two independent methods, indicating that modern satellite instruments can obtain accurate data on moisture over the plateau, which could be usefully assimilated into NWP forecasts (Johnsen et al., 2004[5]).
Atmospheric data derived from ice cores
Temperature proxies
The Antarctic presents a complex picture of temperature change in recent decades (Turner et al., 2005a[6]). While the Antarctic Peninsula and some coastal portions of the Antarctic Ice Sheets have experienced pronounced warming over the last 50 years, most continental stations have shown little or no significant surface warming over this same period. Despite this, significant warming trends have been identified in the mid-troposphere in winter (Turner et al., 2006[7]). (See also Steig et al, 2009[8])
High-resolution (sub-annual) proxy temperature data are derived from stable water isotope measurements on ice cores, and records of such data presently range from several centuries up to around 1,000 years (e.g. Graf et al., 2002[9]; Morgan and van Ommen, 1997[10]; Steig et al., 2005[11]). The largest-scale proxy reconstruction presently available in Antarctica (Schneider et al., 2006[12]) extends the record of temperature changes over the continent (excluding the Peninsula) to 200 years, using ice cores from 5 sites. The proxy data show a long-term increase in temperature of around 0.2°C since the late nineteenth century. This change is in phase with the Southern Hemisphere mean until around 1975, when this primarily interior Antarctic temperature record diverges, showing a very slight cooling trend. The proxy reconstruction and observations reveal significant decadal-scale variability in temperatures associated with variability in the Southern Annular Mode (SAM). This suggests that this surface cooling may, at least in part, reflect recent changes in the SAM (Thompson and Solomon, 2002[13]), a connection also noted by Turner et al. (2005a[6]).
Atmospheric circulation proxies using ice cores
Advances in the sampling and analysis of chemical ion concentrations and stable isotope composition of snow and firn have enabled the collection of multivariate data sets from firn and ice cores, which have increasing application as proxy climate data. Glaciochemical time series in conjunction with accumulation rate time series have been used to unravel the likely moisture sources and atmospheric circulation history for many global locations in the last decade. For a review of glaciochemistry and an introduction to its early use as climate proxy data see Legrand and Mayewski (1997[14]).
Coupled to the advancements in geochemical analyses is the availability of global climate reanalysis data, and instrumental station climate data that have enabled the calibration of ice core proxy climate data, particularly pressure. The two main climate reanalysis data sets are: the US National Centers for Environmental Prediction (NCEP) - National Center for Atmospheric Research (NCAR) climate reanalysis data set that is referred to as the NNR data set (Kalnay et al., 1996[15]; Kistler et al., 1985[16]) available from the US National Oceanic & Atmospheric Administration (NOAA); and ERA-40 climate reanalysis data available from the ECMWF. These data sets contain gridded 6 hourly, daily and monthly data for the full range of climate parameters, from the surface through the atmosphere. The monthly data are of most relevance to the calibration of annual to sub-annually resolved ice core data. Sea-salt aerosols, calcium, nitrate and MS aerosols are the most widely used proxies for atmospheric circulation in the Antarctic region. Examples of the use of these proxies to reconstruct atmospheric circulation and precipitation are given below.
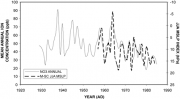
Ice core nitrate as a proxy for atmospheric ridging and the SAM. The Queen Maud and Wilkes Land regions of East Antarctica lie entirely within the strong katabatic wind zone where erosional surface winds from east-southeast to south-southeast drain cold air from the ice sheet's interior down-slope to the coast. Snow precipitation accompanied by an east to east-southeast wind occurs during synoptic-scale maritime cyclonic incursions over the katabatic slope, at least to the 2,000 m elevation. Cullather et al. (1998[17]) established that the strength of the ridging over Wilkes Land influenced the circumpolar storm tracks, resulting in cyclones being steered into Wilkes Land. Murphy and Simmonds (1993[18]) and Bromwich et al. (1993[19]) have established that tropospheric ridging has a significant influence on the surface windfield. Katabatic winds were found to intensify in response to stronger than average surface temperature inversions produced by an enhanced high surface pressure and at the 500 hPa level over the East Antarctic interior. These high pressure anomalies over East Antarctica are associated with a blocking anticyclone to the south east of New Zealand (170 to 180°E), and approaching low pressure systems in the circumpolar trough at 100-120°E. Strong wind events are the product of enhanced katabatic wind flow down slope together with strong geostrophic wind flow across the Wilkes Land slopes (Murphy and Simmonds, 1993[18]). Research by Goodwin et al. (2003[20]) has demonstrated that the nitrate time series from Wilkes Land sites, located in convergent windfields, is a proxy for the strength of the surface windfield. They established from the annual sea salt and nitrate concentration time series that anomalously high accumulation rate and nitrate concentrations at these sites are the product of the accumulation of blown snow, plus increased surface wind speed and/or wind pumping efficiency, rather than synoptic precipitation. Goodwin et al. (2003[20]) have shown that the annual mean nitrate concentration in eastern Wilkes Land snow has a strong statistical relationship with winter (June, July and August) meridional mean sea level pressure (MSLP) indices (Macquarie Island - Scott Base, and Kerguelen Island - Casey MSLP) that describe the oscillations in the SAM (Thompson and Solomon, 2002[13]). The decreasing trend in annual mean nitrate concentration in snow after 1964 suggests a reduced incidence of mid-latitude atmospheric ridging into Wilkes Land during winter (Figure 2.6). This is in agreement with the observed deepening of the circumpolar trough since the mid 1960s (Allan and Haylock, 1993[21]) and the trend towards the high index phase of the SAM (Thompson and Solomon, 2002[13]).
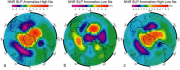
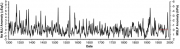
Ice core sea-salt as a proxy for mid-latitude and circum-Antarctic sea-level pressure and the SAM. Goodwin et al. (2004[22]) have derived a 700 year proxy record (at monthly resolution) for winter (May, June, July (MJJ)) MSLP variability over the Southern Ocean, by analysing sea-salt (sodium) aerosol concentrations in the DSS ice core from Law Dome in East Antarctica. The relationship between modern patterns of mid-latitude and sub-Antarctic atmospheric circulation and variations in sodium (Na) delivery to Law Dome ice was identified by analysing co-variations between Na concentrations, MSLP and wind field data. The observed relationship was then used to hindcast a proxy record of early winter MSLP anomalies and the SAM. The proxy mid-latitude MSLP time series is most correlated to atmospheric variability in the region southeast of Australia and south of the Tasman Sea (Macquarie Island and Campbell Island). This is a function of the Rossby Wave number 3 pattern, the periodic atmospheric blocking in this region, and interaction with the SAM. The MSLP patterns (MSLP anomalies that are associated with high and low sea salt aerosol deposition in the DSS (Dome Summit South, Law Dome) core) are shown in Figure 2.7 (Goodwin et al., 2004[22]). The full 700 year record of early winter (MJJ) sea salt sodium and MSLP variability is shown in Figure 2.8 (Goodwin et al., 2004[22]).
Ice core sea-salt as a potential proxy for mid-latitude winter rainfall variability. Recent work by Goodwin (in prep.) has focused on the application of the proxy mid-latitude MSLP and SAM index time series, to investigate methods for hindcasting southern Australian rainfall over the past millennium. Proxy May - July MSLP and SAM data have been cross-correlated against May - July total rainfall data for stations located in southwest and southern Western Australia. Preliminary results, indicate that the highest correlation between the data was calculated for stations close to the coastal escarpment in southwest Western Australia, indicating the strong relationship between winter rainfall, the phase of the SAM and the circumpolar longwave circulation pattern, particularly the meridional location of the Rossby wave number 3 troughs and ridges in the Indo-Australian region. Interdecadal winter rainfall variability across coastal Southern Australia appears to be strongly associated with the time-varying behaviour of the longwave pattern and the SAM. The work in progress indicates that southwest Western Australia experienced periods of higher mean winter rainfall, with high interdecadal variability during 1300 to 1600 AD, followed by lower mean but less variable winter rainfall from 1600 to 1900 AD, which is similar to the past 50 years (Goodwin, in prep.). These preliminary results, illustrate the potential for using high-resolution (monthly) ice core glaciochemical data to reconstruct and predict atmospheric circulation and rainfall distribution patterns across Southern Australia at interannual to interdecadal resolution. Since there is a strong correlation between mid-latitude MSLP, South Indian Ocean sea surface temperatures (SST) and rainfall over southwest Western Australia (Smith I. et al., 2000) ice core sites in Queen Mary Land, East Antarctica appear to have the best potential for reconstructing MSLP and rainfall variability over southwest Western Australia for the past few hundred years. This long record would be of enormous economic benefit to all water users in Western Australia.
Although full-scale reconstructions of past climate over Antarctica have yet to be finalized, SCAR’s International Trans-Antarctic Scientific Expedition (ITASE) (Mayewski et al., 2005a[23]) has, as noted above, pioneered calibration tools and reconstruction of climate indices and evidence for climate forcing using single-sites through to multiple arrays of sites. Initial syntheses of combined ITASE and deep ice core records demonstrate that inclusion of instrumentally calibrated ITASE ice core records allows previously unavailable reconstruction of past regional to continental scale variability in atmospheric circulation and temperature (Mayewski et al., 2005a[23]). Emerging results demonstrate the utilization of ITASE records in testing meteorological reanalysis products (e.g. Genthon et al., 2005[24]). Connections have also been made between ITASE climate proxies and global scale climate indices such as ENSO (Meyerson et al., 2002[25]; Bertler et al., 2004[26]) and to major atmospheric circulation features over the Southern Hemisphere - such as the Amundsen Sea Low, East Antarctic High, Southern Hemisphere westerlies and SAM (Kreutz et al., 2000a[27]; Souney et al., 2002[28]; Goodwin et al., 2004[22]; Proposito et al., 2002[29]; Shulmeister et al., 2004[30]; Kaspari et al., 2005[31]; Yan et al., 2005[32]). ITASE research is also focused on understanding the factors that control natural climate variability over Antarctica and the Southern Ocean, through, for example, documentation of the impact of solar forcing via UV induced changes in stratospheric ozone concentration on the strength of the zonal westerlies at the edge of the polar vortex (Mayewski et al., 2005b[33]).
ITASE proxy circulation reconstructions provide an estimate for the state of modern Antarctic climate. Evidence is emerging for inland penetration of marine tropospheric air masses over the past few decades in summer into portions of coastal West Antarctica near the Amundsen Sea (Dixon et al., 2005[34]) and the Antarctic Peninsula. However, ice core proxy reconstructions for the Amundsen Sea Low suggest that this system is still within the range of variability established over the last 1,200 years (Mayewski and Maasch, 2006[35]), while proxy reconstruction of the Southern Hemisphere westerlies reveals intensification in the 1980s (Dixon et al., in review) consistent with the impact of human-induced changes in stratospheric ozone on the strength of the westerlies around the edge of the polar vortex (Thompson and Solomon, 2002[13]).
Snow Accumulation
Field campaigns traversing many thousands of kilometres have revealed the broad pattern of snow accumulation across the featureless plateau of the ice sheet (the input term in the ice-budget). This can be measured by sampling snow from pits or ice cores then counting seasonal cycles of isotopes, dust, and other chemical impurities (e.g. Mayewski et al., 2005a[23]; Frezzotti et al., 2005[36]; Monaghan et al., 2006a[37]). If the density is also measured, the mass of snow deposited within each year can be found. Repeated observations of snow stakes provide similar information (Kameda et al., 2008[38]; Takahashi and Kameda, 2007[39]). One of the most reliable methods is to detect the layers contaminated by radioactive fallout from atomic bomb tests performed in the 1950s and 1960s (Kamiyama et al., 1989[40]). By measuring the mass of snow that has accumulated above this layer, an unambiguous measurement of snow accumulation is provided.
There are now several thousand observations of snow accumulation across the ice sheet, and these have been compiled into maps of the mean annual snow accumulation rate (Vaughan et al., 1999[41]; Takahashi et al., 1994[42]). Satellites and atmospheric models provide some information about snow accumulation away from traverse routes, and this can help to interpolate the field observations (Giovinetto and Zwally, 2000[43]; Arthern et al., 2006[44]; van de Berg et al., 2006[45]). The published maps are broadly similar in character, showing high coastal accumulation rates and a cold, precipitation-starved interior. The largest differences between estimates are found in the coastal regions where poor weather has hitherto limited the amount of data collected by field parties.
Measurements of snow accumulation have been supplemented by field-based radar. The radar reveals layering within the ice that has been deposited over time. Comparisons with independently dated ice cores have confirmed that bright reflecting layers accurately trace layers of snow that were deposited synchronously, referred to as isochrones. By dating a particular isochrone using an ice core, then following how its depth varies from place to place, the spatial gradients of snow accumulation can be determined (e.g. Richardson-Naslund, 2004[46]; Spikes et al., 2004[47]). Ice penetrating radars are already flown on aircraft, and in the future will be flown on satellites, such as Cryosat-2 (Hawley et al., 2006[48]). These will provide more complete coverage of the stratigraphy of the ice sheet. For a review of accumulation measurements using such methods see Eisen et al. (2008[49]).
When multiple layers are traced, a complete spatio-temporal picture of how snow has accumulated along the traverse route can be established. Surveys of this kind have revealed that the accumulation upon the ice sheet is not uniform, but varies erratically: drifting depletes some locations of snow and provides others with a surfeit. The effect can be especially severe in low accumulation regions where drifting produces dune-like structures (Fahnestock et al., 2000[50]; Frezzotti et al., 2002[51]). The effect of drift makes it difficult to analyse trends in Antarctic snowfall over recent decades. Nevertheless, combining many core records and forming decadal averages gives a measure of how snowfall has varied in Antarctica.
Most of Antarctica is too cold for surface melt to occur, but there is some melting around the periphery. This can be observed from space because the signature of wet snow is quite different from dry snow in the microwave spectrum (Ridley, 1993[52]; Zwally and Fiegles, 1994[53]). This method has been used to study how melt rates vary from year to year. Between 1980 and 2006, melt seasons over the ice shelves of the Antarctic Peninsula lengthened (Torinesi et al., 2003[54]). In the latter decade of this interval (1996 to 2006) melt seasons shortened in the Peninsula, Ronne-Filchner and Amundsen Sea sectors of Antarctica, but lengthened over much of East Antarctica and the Ross Ice Shelf (Torinesi et al., 2003[54]). The pattern of melting generally follows trends in air temperatures (Liu et al., 2006[55]). Snow surface temperature can be measured from space using images from the Advanced Very High Resolution Radiometer (AVHRR), which is sensitive to infra-red radiation emitted by the surface (Comiso, 2000[56]). This temperature information has proved useful in glaciological applications, including modelling snow compaction (Li et al., 2007[57]) and detecting glazed surface (Fujii et al., 1987[58]), which develops on steeper slope of the undulating ice sheet suraface (Furukawa et al., 1996[59]).
Although microwave radar altimeters are designed to measure surface elevation, the backscattered power can also provide information on snow properties (Legresy and Remy, 1997[60]; Arthern et al., 2001[61]). Passive microwave radiometers carried by satellites and scatterometers provide complimentary information on the snow structure and how it changes (Surdyk and Fily, 1994[62]; Sherjal and Fily, 1994[63]; Long and Drinkwater, 1999[64]; Rotschky et al., 2006[65]). It has proved difficult to derive physical and climatic quantities related to snow structure directly from microwave observations, but these satellites can provide spatial information that extends the usefulness of field observations. Microwave observations from the Advanced Microwave Scanning Radiometer (AMSR-E) instrument have been combined with temperatures measured by AVHRR to assist the interpolation of sparsely-spaced observations of snow accumulation rate (Arthern et al., 2006[44]).
The scale of the Antarctic ice sheet, and the difficulties of working there, mean that remote sensing methods using satellites are the only practical way to get an overview of how the entire ice sheet is changing. The distinction between satellite remote sensing and fieldwork is becoming more blurred as field operations are targeted towards important changes identified by satellite and as fieldwork provides calibration and validation measurements for specific satellite campaigns. Although the time series of available satellite observations grows longer each year, it is still a short record compared to observations from the surface. Field-based observations using radar, seismic and ice cores can resolve extra dimensions in depth, and hence time, through the layered structure of the ice sheet. They can also give invaluable insights into what has caused changes that are observed using satellites.
References
- ↑ Farman, J.C., Gardiner, B.G. and Shanklin, J.D. 1985. Large losses of total ozone in Antarctica reveal seasonal ClOx/NOx interaction, Nature, 315, 207-210.
- ↑ 2.0 2.1 Francis, J.A. 1994. Improvements to TOVS retrievals over sea ice and applications to estimating Arctic energy fluxes, Journal of Geophysical Research, 99, 10395-10408.
- ↑ Bromwich, D.H., Fogt, R.L., Hodges, K.I. and Walsh, J.E. 2007. A tropospheric assessment of the ERA-40, NCEP, and JRA-25 global reanalyses in the polar regions, J. Geophys. Res., 112, Art. No. D10111.
- ↑ Lachlan-Cope, T.A. 1992. The use of a simultaneous physical retrieval scheme for satellite derived atmospheric temperaturesL Weddell Sea, Antarctica, International Journal of Remote Sensing, 13, 141-154.
- ↑ Johnsen, K-P., Miao, J. and Kidder, S.Q. 2004. Comparison of atmospheric water vapour overarctica derived from CHAMP/GPS and AMSU-B data, Physics and Chemistry of the Earth, 29, 251-255.
- ↑ 6.0 6.1 Turner, J., Colwell, S.R., Marshall, G.J., Lachlan-Cope, T.A., Carleton, A.M., Jones, P.D., Lagun, V., Reid, P.A. and Iagovkina, S. 2005a. Antarctic climate change during the last 50 years, International Journal of Climatology, 25, 279-294.
- ↑ Turner, J., Lachlan-Cope, T.A., Colwell, S.R., Marshall, G.J. and Connolley, W.M. 2006. Significant warming of the Antarctic winter troposphere, Science, 311, 1914-1917.
- ↑ Steig, E.J., Schneider, D.P., Rutherford, S.D., Mann, M.E., Comiso, J. C. and Shindell, D.T. 2009. Warming of the Antarctic ice-sheet surface since the 1957 International Geophysical Year, Nature, 457, 459-462.
- ↑ Graf, W., Oerter, H., Reinwarth, O., Stichler, W., Wilhelms, F., Miller, H. and Mulvaney, R. 2002. Stable isotope records from Dronning Maud Land, Antarctica, Annals of Glaciology, 35, 195-201.
- ↑ Morgan, V.I. and Van Ommen, T.D. 1997. Seasonality in late-Holocene climate from ice core records, The Holocene, 7, 351-354.
- ↑ Steig, E.J., Mayewski, P.A., Dixon, D.A., Kaspari, S.D., Frey, M.M., Schneider, D.P., Arcone, S.A., Hamilton, G.S., Spikes, V.B., Albert, M., Meese, D., Gow, A.J., Shuman, C.A., White, J.W.C., Sneed, S., Flaherty, J. and Wumkes, M. 2005. High-resolution ice cores from US-ITASE (West Antarctica): Development and validation of chronologies and determination of precision and accuracy, Annals of Glaciology, 41, 77-84.
- ↑ Schneider, D.P., Steig, E.J., Van Ommen, T.D., Dixon, D.A., Mayewski, P.A., Jones, J.M. and Bitz, C.M. 2006. Antarctic temperatures over the past two centuries from ice cores, Geophysics Research Letters, 33, L16707, doi:10.1029/2006GL027057.
- ↑ 13.0 13.1 13.2 13.3 Thompson, D. and Solomon, S. 2002. Interpretation of recent southern hemisphere climate change, Science, 296(5569), 895-899.
- ↑ Legrand, M. and Mayewski, P. 1997. Glaciochemistry of polar ice cores: a review, Rev. of Geophys., 35 (3), 219-243.
- ↑ Kalnay, E. and coauthors. 1996. The NCEP/NCAR 40-year reanalysis project, Bull. Amer. Meteor. Soc., 77, 437-471.
- ↑ Kistler, R. and coauthors. 1985. The NCEP/NCAR 50-year reanalysis project, Bull. Amer. Meteor. Soc., 82, 247-267.
- ↑ Cullather, R.I., Bromwich, D.H. and Van Woert, M.L. 1998. Spatial and temporal variability of Antarctic precipitation from atmospheric methods, J. Climate, 11, 334-367.
- ↑ 18.0 18.1 Murphy, B.F. and Simmonds, I. 1993. An analysis of strong wind events simulated in a GCM near Casey in the Antarctic, Monthly Weather Review, 121, 522-534.
- ↑ Bromwich, D.H., Carrasco, J.F., Liu, Z. and Tzeng, R.T. 1993. Hemispheric atmospheric variations and oceanographic impacts associated with katabatic surges across the Ross Ice Shelf, Antarctica, Journal of Geophysical Research, 98 (D7), 13,045-13,062.
- ↑ 20.0 20.1 Goodwin, I., De Angelis, M., Pook, M. and Young, N.W. 2003. Snow accumulation variability in Wilkes Land, East Antarctica and the relationship to atmospheric ridging in the 130° to 170° E region since 1930, Journal of Geophysical Research, 108, D21, 4673, doi:10.1029/2002JD002995.
- ↑ Allan, R. J. and Haylock, M. R. 1993. Circulation features associated with the winter rainfall decrease in southwestern Australia. Journal of Climate, 6, 1356-1367.
- ↑ 22.0 22.1 22.2 22.3 Goodwin, I.D., Van Ommen, T.D., Curran, M.A.J. and Mayewski, P.A. 2004. Mid latitude winter climate variability in the south Indian and south-west Pacific regions since 1300 AD, Climate Dynamics, 22, 783-794, DOI:10.1007/S00382-004-0403-3.
- ↑ 23.0 23.1 23.2 Mayewski, P.A., Frezzotti, M., Bertler, N., Van Ommen, T., Hamilton, G., Jacka, T.H., Welch, B., Frey, M., Dahe, Q., Jiawen, R., Simöes, J., Fily, M., Oerter, H., Nishio, F., Isaksson, E., Mulvaney, R., Holmund, P., Lipenkov, V. and Goodwin, I. 2005a. The International Trans-Antarctic Scientific Expedition (ITASE): an overview, Ann. Glaciol., 41(1), 180-185.
- ↑ Genthon, C., Kaspari, S. and Mayewski, P.A. 2005. Inter-annual variability of surface mass balance in West Antarctica from ITASE cores and ERA40 reanalyses, Climate Dynamics, 21, DOI10.1007/s00382-003-0329-1.
- ↑ Meyerson, E.A., Mayewski, P.A., Whitlow, S.I., Meeker, L.D., Kreutz, K.J. and Twickler, M.S. 2002. The extratropical expression of ENSO recorded in a South Pole glaciochemical time series, Annals of Glaciology, 35, 430-436.
- ↑ Bertler, N.A.N., Barrett, P.J., Mayewski, P.A., Fogt, R.L., Kreutz, K.J. and Shulmeister, J. 2004. El Niño suppresses Antarctic warming, Geophys. Res. Lett., 31, L15207, doi:10.1029/2004GL020749.
- ↑ Kreutz, K.J., Mayewski, P.A., Pittalwala, I.I., Meeker, L.D., Twickler M.S. and Whitlow, S.I. 2000a. Sea-level pressure variability in the Amundsen Sea region inferred from a West Antarctic glaciochemical record, Journal of Geophysical Research, 105 (D3), 4047-4059.
- ↑ Souney, J., Mayewski, P.A., Goodwin, I., Morgan, V. and Van Ommen, T. 2002. A 700-year record of atmospheric circulation developed from the Law Dome ice core, East Antarctica, J. Geophys. Res., 107 (D22), 4608-4616.
- ↑ Proposito, M., Proposito, M., Becagli, S., Castellano, E., Flora, O., Gragnani, R., Stenni, B., Traversi, R., Udisti, R. and Frezzotti, M. 2002. Chemical and isotopic snow variability along the 1998 ITASE traverse from Terra Nova Bay to Dome C (East-Antarctica), Ann. Glaciol., 35, 187-194.
- ↑ Shulmeister, J., Goodwin, I., Renwick, J., Harle, K., Armand, L., McGlone, M. S., Cook, E., Dodson, J., Hesse, P. P.,Mayewski, P.A. and Curran, M. 2004. The Southern Hemisphere Westerlies in the Australasian sector: A synthesis, Quaternary International, 118-119, 23-53.
- ↑ Kaspari, S., Mayewski, P.A., Dixon, D., Sneed, S.B. and Handley, M.J. 2005. Sources and transport pathways for marine aerosol species into West Antarctica, Annals of Glaciology, 42, 1-9.
- ↑ Yan, Y., Mayewski, P.A., Kang, S. and Meyerson, E. 2005. An ice core proxy for Antarctic circumpolar wind intensity, Annals of Glaciology, 41, 121-130.
- ↑ Mayewski, P.A., Maasch, K., Yan, Y., Kang, S., Meyerson, E., Sneed, S., Kaspari, S., Dixon, D., Morgan, V., Van Ommen, T. and Curran, M. 2005b. Solar forcing of the polar atmosphere, Annals of Glaciology, 41, 147-154.
- ↑ Dixon, D., Mayewski, P.A., Kaspari, S., Sneed, S. and Handley, M. 2005. Connections between West Antarctic ice core sulfate and climate over the last 200+ years, Annals of Glaciology, 41, 155-166.
- ↑ Mayewski, P.A. and Maasch, K. 2006. Recent warming inconsistent with natural association between temperature and atmospheric circulation over the last 2000 years. Climate of the Past (Discussions) http://www.copernicus.org/EGU/cp/cpd/2/327/cpd-2-327.htm.
- ↑ Frezzotti, M. and 13 others 2005. Spatial and temporal variability of snow accumulation in East Antarctica from traverse data. J. Glaciol., 51(172), 113-124.
- ↑ Monaghan, A.J., Bromwich, D.H. and Wang, S-H. 2006a. Recent trends in Antarctic snow accumulation from Polar MM5, Philosophical Trans. Royal. Soc. A, 364, 1683-1708.
- ↑ Kameda, T., Motoyama, H., Fujita, S. and Takahashi, S. 2008. Temporal and spatial variability of surface mass balance at Dome Fuji, East Antarctica, by the stake method from 1995 to 2006, Journal of Glaciology, 54(184), 107-116.
- ↑ Takahashi, S. and Kameda, T. 2007. Snow density for measuring surface mass balance using the stake method, Journal of Glaciology, 53(183), 677-680.
- ↑ Kamiyama, K., Ageta, Y. and Fujii, Y. 1989. Atmospheric and depositional environments traced from unique chemical compositions of the snow over an inland high plateau, Antarcitca, J. Geophys. Res., 94, D15, 18515-18519.
- ↑ Vaughan D.G., Bamber, J.L., Giovinetto, M.B., Russell, J. and Cooper, A.P.R. 1999. Reassessment of net surface mass balance in Antarctica, Journal of Climate, 12, No. 4, 933-946.
- ↑ Takahashi, T., Ageta, Y., Fujii, Y. and Watanabe, O. 1994. Surface mass balance in east Dronning Maud Land, Antarctica, observed by Japanese Antarctic Research Expeditions, Ann. Glaciol, 20, 242-248.
- ↑ Giovinetto, M.B. and Zwally, H.J. 2000. Spatial distribution of net surface accumulation on the Antarctic ice sheet, Ann. Glaciol., 31, 171-178.
- ↑ 44.0 44.1 Arthern, R.J., Winebrenner, D.P. and Vaughan, D.G. 2006. Antarctic snow accumulation mapped using polarization of 4.3-cm wavelength microwave emission, J. Geophys. Res., 111, D06107, doi:10.1029/2004JD005667.
- ↑ Van De Berg, W.J., Van Den Broeke, M.R., Reijmer, C.H. and Van Meijgaard, E. 2006. Reassessment of the Antarctic surface mass balance using calibrated output of a regional atmospheric climate model, J. Geophys. Res., 111, D11104, doi:10.10292005JD006495.
- ↑ Richardson-Naslund, C. 2004. Spatial characteristics of snow accumulation in Dronning Maud Land, Antarctica, Global and Planetary Change, 42 (1 4), 31-43.
- ↑ Spikes, V.B., Hamilton, G.S., Arcone, S.A., Kaspari, S. and Mayewski, P.A. 2004. Variability in accumulation rates from GPR profiling on the West Antarctic Plateau, Ann. Glaciol., 39, 238-244.
- ↑ Hawley, R.L., Morris, E.M., Cullen, R., Nixdorf, U., Shepherd, A.P. and Wingham, D.J. 2006. ASIRAS airborne radar resolves internal annual layers in the dry-snow zone of Greenland, Geophys. Res. Lett., 33, L04502, doi:10.1029/2005GL025147.
- ↑ Eisen, O., Frezzotti, M., Genthon, C., Isaksson, E., Magand, O., Van Den Broeke, M.R., Dixon, D. A., Ekaykin, A., Holmlund, P., Kameda, T., Karlof, L., Kaspari, S., Lipenkov, V.Y., Oerter, H., Takahashi, S. and Vaughan, D.G. 2008. Ground-based measurements of spatial and temporal variability of snow accumulation in east Antarctica, Reviews of Geophysics, 46 (1), doi:10.1029/2006RG000218.
- ↑ Fahnestock, M.A., Scambos, T.A., Shuman, C.A., Arthern, R.J., Winebrenner, D.P. and Kwok, R. 2000. Snow Megadune Fields on the East Antarctic Plateau: Extreme Atmosphere-Ice Interaction, Geophys. Res. Lett., 27(22), 3719 - 3722.
- ↑ Frezzotti, M., Gandolfi, S. and Urbini, S. 2002. Snow megadune in Antarctica: Sedimentary structure and genesis, J. Geophys. Res. 107 (D18) 4344, doi:10.1029/2001JD000673.
- ↑ Ridley, J. 1993. Surface melting on Antarctic peninsula ice shelves detected by passive microwave sensors, Geophys. Res. Lett., 20(23), 2639-2642.
- ↑ Zwally, H.J. and Fiegles, S. 1994. Extent and duration of Antarctic surface melting, J. Glaciol., 40(136), 463-476.
- ↑ 54.0 54.1 Torinesi, O., Fily, M. and Genthon, C. 2003. Variability and trends of summer melt period of Antarctic ice margins since 1980 from microwave sensors, J. Climate, 16(7), 1047-1060.
- ↑ Liu, X-D., Li, H-C., Sun, L-G., Yin, X-B., Zhao, S-P. and Wang, Y-H. 2006. δ13C and δ15N in the ornithogenic sediments from the Antarctic maritime as palaeoecological proxies during the past 2000 yr, Earth and Planetary Science Letters, 243, 424-438.
- ↑ Comiso, J.C. 2000. Variability and trends in Antarctic surface temperatures from in situ and satellite infrared measurements, Journal of Climate, 13, 1674-1696.
- ↑ Li, J., Zwally, H.J. and Comiso, J.C. 2007. Ice-sheet elevation changes caused by variations of the firn compaction rate induced by satellite-observed temperature variations (1982-2003), Ann. Glaciol., 46, 8-13.
- ↑ Fujii, Y., Yamanouchi, T., Suzuki, K. and Tanaka, S. 1987. Comparison of the surface conditions of the inland ice sheet, Dronning Maud Land, Antarctica, derived from NOAA/ AVHRR data with ground observation, Annals of Glaciology, 9, 72-75.
- ↑ Furukawa, T., Kamiayama, K. and Maeno, H. 1996. Snow surface features along the traverse route from the coast to Dome Fuji Station, Queen Maud Land, Antarctica, Proc. NIPR Symp. Polar Meteorol, Glaciol., 10, 13-24.
- ↑ Legresy, B. and Remy, F. 1997. Altimetric observations of surface characteristics of the Antarctic ice sheet, J. Glaciol., 43(144), 265-275.
- ↑ Arthern, R.J., Wingham, D.J. and Ridout, A.L. 2001. Controls on ERS altimeter measurements over ice sheets: Footprint-scale topography, backscatter fluctuations, and the dependence of microwave penetration depth on satellite orientation, J. Geophys. Res., 106(D24), 33,471-33,484.
- ↑ Surdyk, S. and Fily, M. 1994. Results of a stratified snow emissivity model based on the wave approach: application to the Antarctic ice sheet, J. Geophys. Res., 100(C5), 8837-8848.
- ↑ Sherjal, I. and Fily, M. 1994. Temporal variations of microwave brightness temperatures over Antarctica, Ann. Glaciol., 20, 19-25.
- ↑ Long, D.G. and Drinkwater, M.R. 1999. Cryosphere applications of NSCAT data, IEEE Trans. Geosci. Remote Sens., 37(3), 1671-1684.
- ↑ Rotschky, G., Rack, W., Dierking, W. and Oerter, H. 2006. Retrieving Snowpack Properties and Accumulation Estimates from a Combination of SAR and Scatterometer Measurements, IEEE Transactions on Geoscience and Remote Sensing, 44(4), 943-956., doi:10.1109/TGRS.2005.862524.