Marine and terrestrial pollution
- This page is part of the topic Marine biology in the instrumental period
Increasing concern about global changes and environmental protection is promoting international efforts to assess future trends and to mitigate the main causes and effects of climatic and environmental changes. In the last century the economic and industrial development of the Northern Hemisphere has had a dramatic impact on the global environment. Antarctica, the remotest continent in the Southern Hemisphere, has thus become a symbol of the last great wilderness and pristine environment. However, the world’s future population growth and industrial development will occur in countries of the Southern Hemisphere. The rapidly changing global pattern of persistent anthropogenic contaminants and greenhouse gases may reduce the value of Antarctica and the surrounding Southern Ocean for research on evolutionary and ecophysiological processes and as an ideal archive of data for better understanding global processes (Bargagli, 2005[1]).
Antarctica has a very small, non-native population and is protected by natural “barriers” such as the Antarctic Circumpolar Current and the zone of circumpolar cyclonic vortex, which reduce the entry of water and air masses from lower latitudes and the consequent import of propagules and persistent contaminants. Nonetheless, the recurring appearance of the “ozone hole” and the rapid regional warming of the Antarctic Peninsula indicate that Antarctica and the Southern Ocean are inextricably linked to global processes and that they are not escaping the impact of local and global anthropogenic activities.
Exploration, research, sealing and whaling have drawn people to Antarctica since the early 1900s, and the development of research, tourism and fishing in the last 50 years has driven a striking increase in human presence. Local impacts due to the presence of humans, such as contamination of air, ice, soil, marine sediments and biota through fuel combustion (for transportation and energy production), waste incineration, oil spillage and sewage, are inevitable, especially near operational bases. Another serious anthropogenic impact, especially in the sub-Antarctic islands (where climatic and environmental conditions are less extreme) is the introduction of alien organisms (Frenot et al., 2005[2]). Although the number of tourists visiting Antarctica is usually two-three times greater than that of the logistic and scientific personnel, the latter reside for a longer time in permanent or semi-permanent stations. Most stations are located in coastal areas and until twenty years ago refuse was dumped into landfill sites or the sea or burnt in the open air. Several accidental spills of oil, lubricants and foreign chemical compounds have occurred at and around Antarctic stations; unfortunately, in the Southern Ocean there have also been significant oil spills, such as the release in January 1989 of about 550 m3 of diesel fuel during the sinking of the Argentine supply ship Bahia Paraiso, near Anvers Island (Antarctic Peninsula).
Contents
Local-scale pollution
The IGY (1957-1958), with the involvement of 12 countries and over 5,000 persons occupying 55 stations in the continent and the islands of the Southern Ocean, marked the beginning of significant local detrimental impacts on the Antarctic environment. Although concern about local environmental pollution has been expressed since the 1970s (e.g. Cameron, 1972[3]), the value of the Antarctic environment to science was only definitively acknowledged in 1991 with the adoption of the Madrid Protocol to the Antarctic Treaty. The Protocol marked the exclusion of Antarctica from the geopolitics of the Cold War period, territorial claims and the possible exploitation of natural resources. It provided strict guidelines for environmental management and protection, and established the obligation to clean-up abandoned work sites. Some countries began to document environmental pollution at abandoned stations and waste-disposal sites, and developed strategies for clean-up and remediation (e.g., Kennicutt et al., 1995[4]; Deprez et al., 1999[5]; COMNAP-AEON, 2001[6]; Webster et al., 2003[7]; Stark et al., 2006[8]). Trace metals and several Persistent Organic Pollutants (POPs) such as Polycyclic Aromatic Hydrocarbons (PAHs) and other by-products of combustion and incineration processes, including Polychlorinated Dibenzo-p-Dioxins (PCDDs), Polychlorinated Dibenzofurans (PCDFs) and Polychlorinated Biphenyls (PCBs), were among the most common persistent contaminants in terrestrial and marine ecosystems within a few hundred metres of scientific stations (UNEP, 2002[9]). However, despite existence of the Protocol, its translation into practice is patchy at best, and much remains to be achieved even to quantify direct human impacts on the Antarctic, let alone effective mitigation where required (Tin et al., 2009[10]).
Most ice-free areas of continental Antarctica are cold desert environments with sparse biotic communities, comprising few species of microorganisms, cryptogams and invertebrates. Although there are several reports on the distribution of persistent contaminants in Antarctic soils, mosses and lichens (e.g. Bacci et al., 1986[11]; Claridge et al., 1995[12]; Bargagli, 2001[13]), possible long-term (biotic and abiotic) effects of persistent contaminants in Antarctic terrestrial ecosystems are largely unknown. There is evidence that hydrocarbon spillage in soils can result in an increase in hydrocarbon-degrading microbes and concomitant decrease in the diversity of the soil microbial community (Aislabie et al., 2004[14]). However, the “in situ” biodegradation rate is probably very low, because aliphatic and aromatic compounds can be detected in soils more than 30 years after a spill.
In striking contrast with the extremely reduced number of species in terrestrial biotic communities, most Antarctic marine ecosystems have a rich variety of species and a high biomass. Contaminants are introduced in the coastal marine environment through waste water, leachates from dump sites, and deposition of particulates from station activity and ship operations. The accumulation of metals and POPs has been reported in samples of water, sediments and organisms collected in the vicinity of several Antarctic stations (e.g. UNEP, 2002[9]; Bargagli, 2005[1]). Throughout the 1970s, wastes from McMurdo station were routinely discharged along the eastern shoreline of Winter Quarter Bay, which also provided docking facilities for ships. In 1988 the US National Science Foundation began a dumpsite cleanup and abatement programme and the bay became one of the most studied marine environment in Antarctica. Concentrations of PAHs, PCBs, Polichlorinated Terphenyls (PCTs) and metals such as Ag, Pb, Sb and Zn in sediments from the bay were much higher than in samples from outside the area (Risebrough et al., 1990[15]; Lenihan et al., 1990[16]; Kennicutt et al., 1995[4]).
In Antarctica, evolutionary processes in isolation contributed to the development of rich communities of coastal benthic organisms such as sponges, hydroids, tunicates, polychaetes, molluscs, actinarians, echinoderms, amphipods and fish. These organisms are characterized by a high degree of endemism and ecophysiological adaptations to peculiar physico-chemical features of the Southern Ocean. As many species are long-lived, have low metabolic rates, lack the pelagic larval phase and need longer development time, Antarctic benthic organisms are more exposed to the long-term effects of environmental contaminants than are temperate or tropical species. Significant disturbance of benthic communities has generally been reported in the proximity of the most polluted coastal sites (e.g., Lenihan and Oliver, 1995[17]; Conlan et al., 2000[18]; Stark et al., 2003[19]). Organism responses to the combined effects of toxic pollutants and organic enrichment from sewage disposal usually involve a decrease in the abundance and diversity of benthic fauna and an increase in resistant and opportunistic species. Research on polluted sediments near Casey Station (Cunningham et al., 2005[20]) has revealed that benthic diatom communities are good indicators of anthropogenic metal contamination and may be useful in monitoring the success of environmental remediation strategies in polluted Antarctic sites.
In recent years there has been considerable interest in astrobiology and microbial forms living in extreme environments. Antarctica has become one of the most important places for research on bacteria that thrive on ice or in subglacial lakes. One of the main challenges for such research is the contamination of ice, especially by drilling fluids. These fluids are a complex mixture of aliphatic and aromatic hydrocarbons and foranes that coat the ice surface and may penetrate into the interior of the ice through micro-fissures. As a rule, the fluid is not sterilized during use, and Alekhina et al. (2007[21]) isolated bacteria of the genus Sphingomonas (a well-known degrader of polyaromatic hydrocarbons) as well as bacteria attributable to human and soil sources from specimens of the deepest (3,400 and 3,600 m) ice borehole at Lake Vostok.
Global-scale contaminants
Today, human activity is one of the most important parameters affecting the chemical composition of the atmosphere. The most marked recent changes in the chemistry of the atmosphere over Antarctica are associated with greenhouse gases, notably the largely anthropogenically caused decrease in stratospheric ozone, and increases in anthropogenically sourced CO2 (380 ppmv), CH4 (1755 ppbv), and N2O (319 ppbv). Anthropogenically sourced radionuclides stemming from above ground nuclear bomb testing are also present throughout Antarctica, as is evidence of the Chernobyl nuclear accident (Dibb et al., 1990[22]), and have even been used to provide dating control within long-lived biological systems (Clarke, 2008[23]).
Of particular concern also is how to unravel human-induced changes in the atmospheric cycles of heavy metals entering the Antarctic atmosphere. The chemical analysis of snow and ice deposited over time in polar ice caps gives unique information on the pollution of the atmosphere with heavy metals over the last centuries. It should however be emphasized that these Antarctic archives mainly reflect pollution emitted in the Southern Hemisphere, especially in South America and South Africa. This is because pollution emitted in the Northern Hemisphere is less likely to penetrate into the Southern Hemisphere and ultimately to reach Antarctica. Deciphering these frozen archives is very difficult. Antarctic snow is so clean that the utmost precautions are required to collect the samples without contaminating them. As an example, one thousand tonnes of Antarctic snow typically contains no more than a few milligrams of lead. Heavy metals, metals or metalloids associated with contamination and potential toxicity, are derived from both natural and anthropogenic sources. While the main natural sources of heavy metals include rock and soil, volcanoes, sea-salt spray, wild forest fires, and continental and marine biogenic activities (Nriagu, 1989[24]), anthropogenic toxic metals come mainly from industrial and domestic activities such as the mining and smelting of metals, the combustion of fossil fuels and refuse incineration (Nriagu and Pacyna, 1988[25]).
Local emissions of heavy metals from human activities in Antarctica have the potential to give rise to environmental contamination in local areas. Major anthropogenic emissions within Antarctica are related to fuel burning for operation of Antarctic stations, snowmobiles, heavy vehicles, ships and aircraft (Boutron and Wolff, 1989[26]). Although local effects may be confined to an area close to the site of such activities, special attention has to be given to local pollution related to stations and regularly utilized traverse routes (Qin et al., 1999[27], because human activities within Antarctica are increasing in number, magnitude, and intensity. An inventory of these emissions is needed for assessing the discharges of heavy metals into the Antarctic environment.
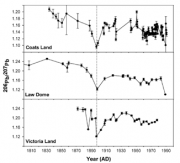
Amongst the most spectacular results obtained so far is the reconstruction of past changes in lead pollution achieved by analyzing a series of snow samples collected in Coats Land, Victoria Land and Law Dome (Van de Velde et al., 2005[28]). Lead isotopic profiles (Figure 4.54) show that lead pollution of Antarctica started as early as the 1880s. This early pollution was at least partially linked with non-ferrous metal production activities in South America, South Africa and Australia. Another important contribution was from coal-powered ships that crossed Cape Horn en route between the Atlantic and Pacific Oceans, as well as whaling ships and shore based stations along the Antarctic coast and Southern Ocean islands. This lead pollution then declined in the 1920s, correlated with the opening of the Panama Canal in 1914, which resulted in a pronounced decrease in ship traffic around the southern tip of South America. Lead pollution then increased again after World War II because of the very large rise in the use of leaded petrol in various countries in the Southern Hemisphere, combined with the continuous increase in non-ferrous metal production in South America, South Africa and Australia. Finally, lead concentrations strongly declined from the mid 1980s onwards, because of the fall in the use of leaded petrol in modern cars.
There is growing evidence from Antarctic snow and ice sampling highlighting the fact that Antarctica is already significantly contaminated with other metals such as Cr, Cu, Zn, Ag, Bi and U as a consequence of long-distance transport from surrounding continents (Wolff and Suttie, 1994[29]; Wolff et al., 1999[30]; Planchon et al., 2002[31]; Vallelonga et al., 2002[32]; Van de Velde et al., 2005[28])
POPs, pesticides and industrial chemicals, are ubiquitous anthropogenic compounds that are released around the world and found in the remote polar regions far distant from their primary sources, due to long-range atmospheric transport. Because they are hydrophobic, persistent and toxic, the trend of their distribution and their concentration are urgently needed to assess environmental contamination. The distribution pattern and levels of POPs such as PCBs and chlorinated pesticides such as Dichlorodiphenyltrichloroethane (DDT) and hexachloribenzene (HCB) are reported in the Antarctic environment (Fuoco et al., 1996[33]; Carsolini et al., 2002[34]; Weber and Goerke, 2003[35]; Montone et al., 2003[36]). Changing levels of some compounds of POPs in living organisms such as fish species reflect global redistribution and increasing transfer to Antarctic waters probably due to recent usage in the Southern Hemisphere and climate change (Weber and Goerke, 2003[35]).
Despite increasing documentation, our ability to quantify environmental contamination from natural and industrial sources and to clarify the effects of various physico-chemical processes controlling atmospheric cycles of anthropogenic pollutants in the Antarctic is still limited because of weaknesses in the available scientific database.
Although some human activity in Antarctica, such as ship or aircraft transportation or the release of weather and research balloons can have widespread effects, scientists, tourists and fishermen are generally the main causes of local disturbance of the Antarctic environment. As pesticides have neither been produced nor applied in the continent, the discovery of DDT and its congeners in Antarctic marine biota in the 1960s and in the environment in the 1970s proved that persistent contaminants in the region come from other continents. Since then, HCB, Hexachlorocycloexanes (HCHs), aldrin, dieldrin, chlordane, endrin, heptachlor and other POPs have all been detected in Antarctica and the Southern Ocean. These chemicals are persistent, hydrophobic and lipophilic, accumulate in organisms and biomagnify in marine food chains (UNEP, 2002[9]). While early ecotoxicological studies concentrated mainly on eggshell thickness and reproductive potential of birds, more recent evidence suggests that several POPs are also immunotoxic, endocrine disrupters and tumor promoters.
Although migrating species of marine birds and mammals may contribute to the southward transfer of POPs, their transport to the Southern Ocean and Antarctica mainly occurs through atmospheric and marine pathways. The Antarctic atmosphere loses more heat by radiative cooling than it gains by surface energy exchange and the deficit is balanced by atmospheric transport of heat, gases, moisture and aerosols from lower latitudes. The global transport of radiatively important trace gases such as CO2, CH4 and CFCs contribute for instance, to climate change and springtime ozone depletion events. Particles and reactive gases in air masses are partly removed by cyclonic storms in the belt of “westerlies” and are deposited in the Southern Ocean (Shaw, 1988[37]). However, during the austral summer the circumpolar vortex disappears and long-term records of mineral dust, black carbon and 210Pb at the South Pole and at some coastal Antarctic stations indicate an enhanced poleward transport of air masses (Wolff and Cachier, 1998[38]). For instance, the contamination of Antarctic aerosol and snow by Pb and Cu has been widely documented (e.g., Barbante et al., 1998[39]) and has usually been attributed to leaded petrol, non-ferrous metal mining and smelting and other anthropogenic sources in South America, Africa and Australia. Moreover, although during the last decades the deposition of Pb in Antarctic snow is decreasing, that of Cu, Zn and other elements is increasing (Planchon et al., 2002[31]).
As the two hemispheres of the Earth have separate circulation systems through much of the atmosphere, there is probably a limited input of anthropogenic contaminants from the Northern Hemisphere to Antarctica. However, the profiles of radioactive debris deposition in Antarctic snow and ice during the 1950s and 1960s showed the presence of fission products released in the Northern Hemisphere (Koide et al., 1979[40]). Under ambient temperatures POPs may volatilize from water, soils and vegetation into the atmosphere, where they are unaffected by breakdown reactions and may be transported over long distances before re-deposition. Volatilization/deposition cycles may be repeated many times and according to the theory of cold condensation and global fractionation (Wania and Mackay, 1993[41]) the most volatile compounds such as HCHs, HCB and low-chlorinated PCBs can redistribute globally. Although their concentrations in Antarctic biota are usually below those documented to have reproductive effects in related species in temperate and Arctic regions, organisms living under harsh Antarctic conditions may be more stressed and more vulnerable to the adverse effects of pollutants than those in temperate regions (Bonstra, 2004[42]; Corsolini et al., 2006[43]). There is evidence that in some Antarctic marine organisms concentrations of Cd, Hg and other pollutants can be relatively high (e.g., Bargagli, 2001[13]; Bustnes et al., 2007[44]); furthermore, POP accumulation in marine sediments is increasing their availability and accumulation in benthic fish species feeding on benthic invertebrates (Goerke et al., 2004[45]).
While there is serious concern related to protection of the Antarctic environment, major efforts in international legislation that will help to safeguard the Antarctic environment are underway. The Stockholm Convention on Persistent Organic Pollutants (2004) is a global treaty designed to protect the environment from chemicals that remain intact in the environment for long periods, such as POPs. This legislation follows in the footsteps of international legislation that protects Antarctica such as the Montreal Protocol (1989). The Montreal Protocol is designed to protect the ozone layer by phasing out the production of a number of substances believed to be responsible for ozone depletion.
Concern about the future impact of human activity
New classes of global pollutants are emerging, such as perfluorinated compounds (PFCs) that have a wide range of industrial applications. These compounds have been shown to be toxic to several species of aquatic organisms and to occur in biota from various seas and oceans, including the Arctic and the Southern Ocean (Yamashita et al., 2005[46]). Catalytic converters in motor vehicles are increasing global emissions of Pt and other companion elements such as Pd, Rh, Ru, Os and In. Increased concentrations of Rh, Pd and Pt with respect to ancient Greenland ice samples were measured in surface snow from the Alps, Greenland and Antarctica (Barbante et al., 1999[47]).
Climate change and global warming could enhance the transport and deposition of persistent contaminants in Antarctica. The oceanic transport of persistent contaminants is often considered to be much less important than atmospheric transport; however, models which combine the transport of semi-volatile chemicals in air and water, and consider continuous exchange between the two compartments indicate that the overall transport of POPs to remote regions is accelerated with respect to models treating air and water separately (Beyer and Matthies, 2001[48]). The rapid regional climatic warming of the Antarctic Peninsula has also been detected in oceanic waters to the west (e.g. Meredith and King, 2005[49]). The warming of surface water can affect POP volatilization and transport. In contrast to organisms in temperate and tropical seas, those in the Southern Ocean are well adapted to narrow ranges of water temperature close to the freezing point. Slight increases in temperature may have disproportionate influence on the properties of cell membranes and biological processes involved in the uptake and detoxification of environmental pollutants.
Although for continental Antarctica there is as yet no significant trend in meteorological temperature, a loss of ice shelves such as that in the Antarctic Peninsula (six ice shelves have largely disappeared in the last 50 years) could have dramatic effects on atmospheric precipitation (i.e. the deposition of contaminants) and the biogeochemical cycle of trace elements such as Hg. Mercury emitted by anthropogenic and natural sources occurs in the atmosphere mostly in the gaseous elemental form (Hg0), which has a long lifetime in tropical and temperate regions. Once deposited in terrestrial and aquatic ecosystems the metal is partly re-emitted into air, thus assuming the characteristics of global pollutants such as POPs. The metal is now acknowledged to be one of the most serious contaminants in polar ecosystems because of the springtime Hg depletion events that have been reported in the high Arctic (Schroeder et al., 1998[50]) and Antarctica (Ebinghaus et al., 2002[51]). In polar regions, after sunrise, the globally-distributed Hg0 undergoes photochemically-driven oxidation by reactive halogen radicals (from sea-salt aerosols) and rapidly deposits on snow and on terrestrial and marine ecosystems. Field evidence of enhanced Hg accumulation in soil and cryptogamic organisms from terrestrial ecosystems facing the Terra Nova Bay coastal polynya raises concern that Antarctica may become an important sink in the global Hg cycle (Bargagli et al., 2005[52]). By changing the sea ice cover and increasing the availability of reactive halogens, warming could enhance the role of Antarctica as a “cold trap” for Hg through an increase in the out-gassing of the metal from continents and oceans. Furthermore, while the use of Hg and many POPs has declined or ceased in North America and Europe since the 1990s and earlier, the growing demand for energy, the burning of coal and biomass, the extraction of gold, intensive agriculture, the spraying of pesticides for disease vector control, and the lack of emission control technologies in South America, Africa and Asia will likely increase the atmospheric burden of Hg and many other persistent contaminants.
References
- ↑ 1.0 1.1 Bargagli, R. 2005. Antarctic Ecosystems: Environmental Contamination, Climate Change, and Human Impact. Sprnger-Verlag, Berlin, 395 pp.
- ↑ Frenot, Y., Chown, S.L., Whinam, J., Selkirk, P., Convey, P., Skotnicki, M. and Bergstrom, D. 2005 Biological invasions in the Antarctic: extent, impacts and implications, Biological Reviews, 80, 45-72.
- ↑ Cameron, R.E. 1972. Pollution and conservation of the Antarctic terrestrial ecosystems. In: Parker BC (ed) Proc Coll Conservation Problems in Antarctica. Allen Press, Lawrence, Kansas, 267-305.
- ↑ 4.0 4.1 Kennicutt, M.C., McDonald, S.J, Sericano, J.L., Boothe, P., Oliver, J., Safe, F., Presley, B.J., Liu, H., Wolfe, D., Wade, T.L., Crockett, A., Bockus, D. 1995. Human contamination of the marine environment: Arthur Harbor and McMurdo Sound, Antarctica, Environ. Sci. Technol., 29, 1279-1287.
- ↑ Deprez, P.P., Arens, M. and Locher, E. 1999. Identification and assessment of contaminated sites at Casey Station, Wilkes Land, Antarctica, Polar Record, 35, 299-316.
- ↑ COMNAP-AEON. 2001. Summary and environmental monitoring activities in Antarctica. Council of Managers of National Antarctic Programs, Hobart.
- ↑ Webster, J., Webster, K., Nelson, P. and Waterhouse, E. 2003. The behaviour of residual contaminants at a former station site, Antarctica, Environ. Pollut., 123,163-179.
- ↑ Stark, J.S., Snape, I. and Riddle, M.J. 2006. Abandoned Antarctic waste disposal sites: monitoring remediation outcomes and limitations at Casey Station, Ecol. Manage. Rest., 7, 21-31 .
- ↑ 9.0 9.1 9.2 UNEP 2002. Regionally based assessment of persistent toxic substances – Antarctica regional report. United Nations Environmental Programmme, Chemicals, Geneva.
- ↑ Tin, T., Fleming, Z., Hughes, K.A., Ainley, D., Convey, P., Moreno, C., Pfeiffer, S., Scott, J., and Snape, I. 2009. Impacts of local human activities on the Antarctic environment: a review, Antarctic Science. (in press),
- ↑ Bacci, E., Calamari, D., Gaggi, C., Fanelli, R., Focardi, S. and Morosini, M. 1986. Chlorinated hydrocarbons in lichen and moss samples from the Antarctic Peninsula, Chemosphere, 15, 747-754.
- ↑ Claridge, G.G.C., Campbell, I.B., Powell, H.K.J, Amin, Z.H. and Balks, M.R. 1995. Heavy metal contamination in some soils of the McMurdo region, Antarctica, Antarct. Sci., 7, 9-14.
- ↑ 13.0 13.1 Bargagli, R. 2001. Trace metals in Antarctic organisms and the development of circumpolar biomonitoring networks, Rev Environ Contam Toxicol, 171, 53-110.
- ↑ Aislabie, J.M., Balks, M.R., Foght, J.M. and Waterhous, E.J. 2004. Hydrocarbon spills on Antarctic soils: effects and management. Environ Sci Technol., 38, 1265-1274.
- ↑ Risebrough, R.W., De Lappe, B.W. and Younghans-Haug, C. 1990. PCB and PCT contamination in Winter Quarters Bay, Antarctica, Mar. Pollut. Bull., 21, 523-529.
- ↑ Lenihan, H.S., Oliver, J.S., Oakden, J.M. and Stephenson, M.D. 1990. Intense and localized benthic marine pollution around McMurdo Station, Antarctica, Mar. Pollut. Bull. 21, 422-430 .
- ↑ Lenihan, H.S. and Oliver, J.S. 1995. Anthropogenic and natural disturbance to main benthic communities in Antarctica, Ecol. Appl. 5, 311-326.
- ↑ Conlan, K.E., Rau, G.H., McFeters, G.A. and Kvitek, R.G. 2000. Influence of McMurdo Station sewage on Antarctic marine benthos: evidence from stable isotopes, bacteria, and sewage on Antarctic marine benthos: evidence from stable isotopes, bacteria, and biotic indices. In: Davison W, Howard-Williams C, Broady P (eds) Antarctic Ecosystems: Models for Wider Ecological Understanding. Caxton Press, Christchurch, 315-318.
- ↑ Stark, J.S., Snape, I. and Riddle, M.J. 2003. The effects of petroleum hydrocarbon and heavy metal contamination of marine sediments on recruitment on Antarctic soft-bottom assemblages: a field experimental investigation, J. Exp. Mar. Biol. Ecol., 283, 21-50 .
- ↑ Cunningham, L., Raymond, B., Snape, I. and Riddle, M.J. 2005. Benthic diatom communities as indicators of anthropogenic metal contamination at Casey Station, Antarctica, J. Paleolimnol., 33, 499-513.
- ↑ Alekhina, I.A., Marie, D., Petit, J.R., Lukin, V.V., Zubkov, V.M. and Bulat, S.A. 2007. Molecular analysis of bacterial diversity in kerosene-based drilling fluid from the deep ice borehole at Vostok, East Antarctica. FEMS Microbiol. Ecol., 59, 289-299.
- ↑ Dibb, J., Mayewski, P.A., Buck, C.F. and Drummey, S.M. 1990. Beta radiation from snow, Nature, 344 (6270), 25.
- ↑ Clarke, L.J. 2008. Resilience of the Antarctic moss Ceratodon purpureus to the effects of elevated UV-B radiation and climate change. PhD thesis, U. Wollongong; L.S. Peck and T. Brey 1996, Nature, 380, 207-208.
- ↑ Nriagu, J.O., 1989. A global assessment of natural sources of atmospheric trace metals, Nature, 338, 47-49.
- ↑ Nriagu, J.O. and Pacyna, J.M. 1988. Quantitative assessment of worldwide contamination of air, water and soils by trace metals, Nature, 333, 134-139.
- ↑ Boutron, C.F. and Wolff, E.W., 1989. Heavy metal and sulphur emissions to the atmosphere from human activities in Antarctica, Atmospheric Environment, 23, 1669-1675.
- ↑ Qin, D., Mayewski, P.A., Lyons, W.B., Sun, J. and Hou, S. 1999. Lead pollution in Antarctic surface snow revealed along the route of the International Trans-Antarctic Expedition (ITAE), Ann. Glaciol., 29, 94-98.
- ↑ 28.0 28.1 28.2 Van De Velde, K., Vallelonga, P., Candelone, J.-P., Rosman, K.J.R., Gaspari, V., Cozzi, G., Barbante, C., Udisti, R., Cescon, P. and Boutron, C.F. 2005. Pb isotope record over one century in snow from Victoria Land, Antarctica, Earth and Planetary Science Letters, 232, 95-108.
- ↑ Wolff, E.W. and Suttie, E.D., 1994. Antarctic snow record of Southern Hemisphere lead pollution, Geophysical Research Letters, 21, 781-784.
- ↑ Wolff, E.W., Suttie, E.D. and Peel, D.A. 1999. Antarctic snow record of cadmium, copper and zinc content during the twentieth century, Atmospheric Environment, 33, 1535-1541.
- ↑ 31.0 31.1 Planchon, F.A.M., Boutron, C.F., Barbante, C., Cozzi, V., Gaspari, V., Wolff, E.W., Ferrari, C.P., Cescon, P. 2002. Changes in heavy metals in Antarctic snow from Coats Land since the mid-19th to the late-20th century, Earth and Planetary Science Letters, 200, 207-222.
- ↑ Vallelonga, P., Van De Velde, K., Candelone, J.-P., Morgan, V.I., Boutron, C.F. and Rosman, K.J.R. 2002. The lead pollution history of Law Dome, Antarctica, from isotopic measurements on ice cores: 1500 AD to 1989 AD, Earth and Planetary Science Letters, 203, 291-306.
- ↑ Fuoco, R., Colombini, M. P., Ceccarini, A. and Abete, C. 1996. Polychlorobiphenyls in Antarctica, Microchemical Journal, 54, 384-390.
- ↑ Carsolini, S., Romeo, T., Ademollo, N., Greco, S. and Focardi, S. 2002. POPs in key species of marine Antarctic ecosystem, Microchemical Journal, 73, 187-193.
- ↑ 35.0 35.1 Weber, K. and Goerke, H. 2003. Persistent organic pollutants (POPs) in Antarctic fish: levels, patterns, changes, Chemosphere, 53, 667-378.
- ↑ Montone, R.C., Taniguchi, S. and Weber, R.R. 2003. PCBs in the atmosphere of King George Island, Antarctica, The Science of the Total Environment, 308, 167-173.
- ↑ Shaw, G.E. 1988. Antarctic aerosol: a review, Rev. Geophys., 26, 89-112.
- ↑ Wolff, E.W. and Cachier, H. 1998. Concentrations and seasonal cycle of black carbon in aerosol at a coastal Antarctic station, J. Geophys. Res., 103(D9), 11033-11042, 10.1029/97JD01363.
- ↑ Barbante, C., Turetta, C., Gambaro, A., Capodaglio, G. and, Scarponi, G. 1998. Sources and origins of aerosols reaching Antarctica as revealed by lead concentrations profiles in shallow snow, Ann. Glaciol., 27, 674-678.
- ↑ Koide, M., Goldberg, E.D., Herron, M.M. and Langway, C.C. 1979. Deposition history of artificial radionuclides in the Ross Ice Shelf, Antarctica, Earth Planet Sci. Lett., 44, 205-223.
- ↑ Wania, F. and Mackay, D. 1993. Global fractionation and cold condensation of volatile organochlorine compounds in polar regions, Ambio, 22, 10-18.
- ↑ Bonstra, R. 2004. Coping with changing northern environments: the role of the stress axis in birds and mammals, Integr. Comp. Biol., 4, 95-108.
- ↑ Corsolini, S., Covaci, A., Ademollo, N., Focardi, S., Schepens, P. 2006. Occurrence of organochlorine pesticides (OCPs) and their enantiometric signature, and concentrations of polybrominated diphenyl ethers (PBDEs) in the Adélie penguin food web, Antarctica, Environ. Pollut., 140, 371-382.
- ↑ Bustnes, J.O., Tveraa, T., Varpe, Ø., Henden, J.A. and Skaare, J.U. 2007. Reproductive performance and organochlorine pollutants in an Antarctic marine top predators: the south polar skua, Environ. Int., 33, 911-918.
- ↑ Goerke, H.., Weber, K., Bornemann, H., Ramdohr, S. and Plötz, J. 2004. Increasing levels and biomagnification of persistent pollutants (POPs) in Antarctic biota, Mar. Pollut. Bull., 48, 295-302.
- ↑ Yamashita, N., Kannan, K., Taniyasu, S., Horii, Y., Petrick, G. and, Gamo, T. 2005. A global survey of perfluorinated acids in oceans, Mar. Pollut. Bull., 51, 658-668.
- ↑ Barbante, C., Cozzi, G., Capodaglio, G., Van De Velde, K,, Ferrari, C., Veyssery, A., Boutron, C.F., Scarponi, G. and Cescon, P. 1999. Determination of Rh, Pd, and Pt in polar and Alpine snow and ice by double-focusing ICP-MS with microcentric nebulization, Anal. Chem., 71, 4125-4133.
- ↑ Beyer, A. and Matthies, M. 2001. Long-range transport potential of semivolatile organic chemicals in coupled air-water systems, Environ. Sci. Pollut. Res., 8,173-179
- ↑ Meredith, M.P. and King, J.C. 2005. Rapid climate change in the ocean west of the Antarctic Peninsula during the second half of the 20th century, Geophys. Res. Lett., 32, L19604. (doi: 10.1029/2005GL024042)
- ↑ Schroeder, W.H., Anlauf, K.G., Barrie, L.A., Lu, J.Y., Steffen, A., Schneeberger, D.R. and Berg, T. 1998. Arctic springtime depletion of mercury, Nature, 394, 331-332.
- ↑ Ebinghaus, R., Kock, H.H., Temme, C., Einax, J.W., Richter, A., Burrows, J.P. and Schroeder, W.H. 2002. Antarctic springtime depletion of atmospheric mercury, Environ. Sci. Technol., 36, 1238-1244.
- ↑ Bargagli, R., Agnorelli, C., Borghini, F. and Monaci, F. 2005. Enhanced deposition and bioaccumulation of mercury in Antarctic terrestrial ecosystems facing a coastal polynya, Environ. Sci. Technol., 39, 8150-8155.