Antarctic deglaciation and its impact on global sea level
- This page is part of the topic The last million years
As the Antarctic Ice Sheet retreated its meltwaters contributed to an increase in global sea level. This process continues today and is set to accelerate under predicted increases in temperature. However, in 2007 the Intergovernmental Panel on Climate Change noted continued uncertainty about the exact future contribution of the Earth’s major ice sheets to sea level (IPCC, 2007[1]). The Antarctic Ice Sheet has least evidence to constrain its past volumes and it is not known if in future net loss of ice mass could occur with dynamical ice discharge, rather than surface melting, dominating the ice sheet mass balance. This is important, as better understanding of how global ice sheets have contributed to sea level across Termination I and into the Holocene is one key to predicting how relatively subtle changes in these ice sheets could influence sea level in the future in a warming world. This is especially relevant as evidence from both Antarctica and Greenland show that in the last Eemian (MIS5e) interglacial when temperatures were between 2-5ºC higher global sea levels were 4-6 m higher than today’s (Jansen et al., 2007[2]). When global continental ice sheets grew before the LGM, global sea level was drawn down (Yokoyama et al., 2001[3]). At the end of the last glacial, deglaciation caused sea levels to rise again over a period spanning more than 10 ka. The conventional estimate is that this sea level rise was 120 m (Peltier, 2002[4]), but other evidence suggests that it may have been as much as 130-135 m (Yokoyama et al., 2001[3]). A hundred metres or so was accounted for by the melting of the Northern Hemisphere ice sheets, as indicated by ice sheet models and geological data (Clark et al., 2002[5]). This left the Antarctic Ice Sheet, together with other smaller ice sheets, to supply the rest of the water. At present there are relatively few geological constraints on the extent and thickness of the Antarctic Ice Sheet during and after the LGM and its contribution to global sea level. Most models predict the Antarctic Ice Sheet to have contributed c. 20 m to global sea level rise with estimates ranging from as much as 37 m (Nakada and Lambeck, 1988[6]) to 6-13 m (Bentley, 1999[7]). More recently, an improved three-dimensional thermomechanical model has suggested a contribution of 14-18 m (Huybrechts, 2002[8]). This variability is significant, because when the calculated contributions of the LGM global ice sheets to sea level rise are added together 20% of the water cannot be attributed to any known former ice mass. This is known as the ‘missing water’ (Andrews, 1992[9]). Another uncertainty concerns the ice masses that contributed to meltwater pulse 1a (MWP 1a), a c. 25 m jump in global sea level dated to c. 14.2 ka BP when peak rates of sea level rise potentially exceeded 50 mm/year, equivalent to the addition of 1.5 to 3 Greenland Ice Sheets to the oceans during a period of one to five centuries (Bard et al., 1996[10]). This rapid meltwater event has been variously attributed via modelling studies to the Antarctic Ice Sheet (Clark et al., 2002[5]; Weaver et al., 2003[11]), or the Laurentide and Fennoscandanavian Ice Sheets (Peltier, 2005[12]). Geological evidence does not rule out a contribution from Antarctica (Verleyen et al., 2005[13]; Bassett et al., 2007[14]). Resolving the debates about the Antarctic contribution to global sea level rise and whether or not the Antarctic contributed to Meltwater Pulse 1a has been hampered by a lack of geological evidence or observational control on Antarctic ice thickness and maximum marine limits during the LGM (Huybrechts, 2002[8]).
One method of providing this observational or geological control is to study the history of relative sea level change. This provides one of the primary data sets for constraining the geometry, volume and melt history of past and present glacial masses. These data can be used to determine the loading and rebound of the continental plates under the increasing weight of a growing ice sheet and the decreasing mass of melting ice sheets, and can be used by modellers to indirectly infer former regional ice sheet thickness. Relative sea level (RSL) is the movement of the shoreline relative to the sea. It is influenced by changes in both global ocean volume (eustatic changes in sea level) and local vertical movements of the land (isostatic changes) that are caused by the rebound of the Earth’s crust following the removal of the overlying ice mass. Sometimes, the interaction of these two factors can result in complex curves of RSL change over time, and in other cases they can be influenced by tectonic processes. Typically, these curves are determined for the period since the LGM. Depending on the location of a particular site with respect to former ice masses a number of different shapes of curves can result. For example, in the equatorial regions far from the former ice sheets the RSL curve is dominated by the eustatic component of meltwater returning to the oceans and so shows relative sea level rise. These regions are known as far-field sites. In contrast, at a (near-field) site close to the centre of a former ice sheet mass the RSL change will be dominated by the isostatic component as the crust is unloaded and the land rebounds. If significant ice is removed, and quickly enough, then this rebound is sufficient to outpace the eustatic contribution and so there is a continuous fall in relative sea level like that seen in areas such as Hudson Bay or Sweden today.
Determining RSL change over the Holocene is important because the RSL record provides information on former ice thicknesses in specific regions and on the timing of deglaciation. Crucially, RSL can be determined independently of other glacial geological techniques in two main ways: by using techniques relying on sampling raised marine landforms (beaches, deltas etc), and techniques relying on isolation basins. In the former case, raised marine features are sampled for organic material such as shells, seal skin, penguin remains, or whalebone that can provide an age estimate or constraining date for the age of the beach. For example, whalebone or shells are usually deposited at or just below sea level but over millennia can be reworked into lower beaches and so can only be used as maximum estimates for the age of the beach in which they are found. In the case of isolation basins, cores are taken from lakes near sea level along the coastal margin. Prior to deglaciation, and depending on their altitude, these lakes may have been former marine inlets or basins. As the ice melted and the crust rebounded they became isolated and transformed into freshwater lakes. The sediment in the lakes records this transition, which can be dated to determine when the basin was at sea level. This approach can be used for a staircase of lakes at different altitudes, allowing reconstruction of an RSL curve that is more precise than can be achieved using the raised beach approach.
RSL curves have been developed for many formerly glaciated regions, but those in Antarctica have required the development of some innovative dating techniques. In particular penguin remains and sealskin have been important for constraining the ages of beaches (Baroni and Orombelli, 1994[15]’ Hall et al., 2003[16]; Bentley et al., 2005a[17]).
Spatial coverage of relative sea level (RSL) curves
To date, almost all Antarctic RSL data come from three areas: the Ross Sea sector, the Antarctic Peninsula, and East Antarctic coastal ice-free oases located between 75-105º E and 30-45º E. There are three main reasons for this restricted distribution. First, much of the Antarctic coast (> 95%) is fronted by glacier ice or ice shelves and so there are few sites where RSL change is recorded in coastal sediments. Second, logistical and cost considerations mean that some locations have not yet been sampled for RSL work. Finally, although there is a need for tight dating of RSL data, some sites with raised marine deposits yield little or no organic material suitable for radiocarbon dating.
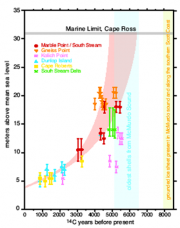
In the Ross Sea area, well-dated RSL curves have been developed from the Scott Coast and Victoria Land Coast (Hall et al., 2003[16]) (Figure 3.16). The Ross Sea curves show exponential RSL fall owing to deglaciation of the coast by the retreating West Antarctic Ice Sheet (Conway et al., 1999[18]). The amount of RSL fall (~ 30 m) is low compared to other formerly glaciated regions (e.g. 150 m for the Canadian Arctic). This is because much of the rebound occurred as restrained rebound, when the coast was still covered by thinning ice or an ice shelf and so no beaches were formed, and the Antarctic ice sheet still continues to provide a substantial load on the crust, unlike the near-full deglaciation of the Canadian Arctic.
Zwartz et al. (1998[19]) and Verleyen et al. (2005[13]) determined RSL curves for the last 15 ka for the Vestfold Hills and Larsemann Hills in East Antarctica by dating a series of marine-lacustrine transitions in isolation basins. The curve is typical of sites close to the margin of formerly more expanded ice sheets in that it shows a RSL rise to a maximum, or ‘highstand’, followed by gradual fall to the present day, athough in the Larsemann Hills there is a decline in the rate of isostatic uplift between c. 7250–6950 and 2847–2509 cal yr BP, due to a mid-Holocene glacier readvance. The RSL highstand in the Larsemann Hills reached approximately +8 m between c. 7570–7270 and 7250–6950 cal yr BP. This shape occurs because the amount of glacio-isostatic rebound at the East Antarctic ice margin in the Early Holocene was sufficiently small that eustatic sea level rise was able to outpace it. When the northern ice sheets had melted and eustatic rise slowed then isostatic rebound outpaced the eustatic signal, leading to a slow RSL fall.
Bentley et al. (2005a[17]) developed the first RSL curves for the Antarctic Peninsula using a combination of morphological remains and isolation basin techniques. In the north, a preliminary RSL curve from the South Shetland Islands shows a similar shape to that from the Larsemann Hills (Verleyen et al., 2005[13]). Here, the highstand reaches ~+14.5-16 m at about 4,000 14C yr BP. In Marguerite Bay (south-central Peninsula) the RSL curve shows exponential RSL fall since deglaciation at ~ 9,000 14C yrs BP. The curve reflects the removal of a large grounded ice sheet that extended across Marguerite Bay to close to the shelf edge (Ó Cofaigh et al., 2002[20]). Both sites are currently the subject of further studies.
Because they reflect former ice volume changes, RSL curves are central to a number of ongoing important debates. These include determining the size of the Antarctic Ice Sheets at the LGM (Bentley, 1999[7]); testing the hypothesis that Meltwater Pulse 1A was wholly or partly derived from Antarctica (Bassett et al., 2007[14]); and determining the timing and style of deglaciation around the ice sheet margin (Conway et al., 1999[18]). One of the most important applications of existing and new RSL curves is to constrain coupled models of ice sheet volume change and glacial-isostatic adjustment. These models attempt to infer reasonable ice sheet histories by testing which histories can satisfy the constraints provided by far-field and near-field RSL datasets. Initial modelling studies have yielded promising results on former ice sheet volume (Nakada et al., 2000[21]; Ivins and James, 2005[22]) and presence/absence of meltwater pulses (Bassett et al., 2007[14]), but the limited distribution of Antarctic RSL sites means that the models are still not as well constrained as for other continents.
Work on developing new RSL curves, particularly using isolation basin techniques, is ongoing at a number of sites. The long-term aim is to improve both the precision and spatial coverage. There are a small number of regions where there are sites suitable for developing RSL curves. They are generally in logistically challenging locations, but the need to improve the spatial coverage of sites in order to better constrain models provides a strong driver for their development.
References
- ↑ IPCC 2007. Climate Change 2007: The Physical Science Basis. Contribution of the Intergovernmental Panel on Climate Change. Cambridge University Press, Cambridge.
- ↑ Jansen, E.J., Overpeck, K.R., Briffa, J-C., Duplessy, F., Joos, V., Masson-Delmotte, D., Olago, B., Otto-Bliesner, W.R., Peltier, S., Rahmstorf, R., Ramesh, D., Raynaud, D., Rind, O., Solomina, R., Villalba, R. and Zhang, D. 2007. Palaeoclimate. In: Solomon SD, Qin M, Manning Z, Chen M, Marquis KB, Averyt M, Tignor, Miller HL (eds) Climate Change 2007: The Physical Science Basis. Contribution of Working Group I to the Fourth Assessment Report of the Intergovernmental Panel on Climate Change. Cambridge University Press, Cambridge, United Kingdom and New York, NY, USA, 433-497.
- ↑ 3.0 3.1 Yokoyama, Y., Lambeck, K., De Deckker, P., Johnston, P. and, Fifield, I.K. 2001. Timing of the Last Glacial Maximum from observed sea-level minima, Nature, 412, 99-119.
- ↑ Peltier, W.R. 2002. Comments on the paper of Yokoyama et al. (2000), entitled Timing of the Last Glacial Maximum from observed sea level minima, Quaternary Science Reviews, 21, 409-414.
- ↑ 5.0 5.1 Clark, P.U., Mitrovica, J.X., Milne, G.A. and Tamisiea, M.E. 2002. Sea-level fingerprinting as a direct test for the source of global meltwater pulse IA, Science, 295, 2438-2441.
- ↑ Nakada, M. and Lambeck, K. 1988. The melting history of the Late Pleistocene Antarctic ice-sheet, Nature, 333, 36-40.
- ↑ 7.0 7.1 Bentley, M.J. 1999. Volume of Antarctic Ice at the Last Glacial Maximum, and its impact on global sea level change, Quaternary Science Reviews, 18, 1569-1595.
- ↑ 8.0 8.1 Huybrechts, P. 2002. Sea-level changes at the LGM from ice-dynamic reconstructions of the Greenland and Antarctic ice sheets during the glacial cycles, Quaternary Science Reviews, 21, 203-231.
- ↑ Andrews, J.T. 1992. Glaciation - a case of missing water. Nature, 358, 281-281.
- ↑ Bard, E., Hamelin, B., Arnold, M., Montaggioni, L., Cabioch, G., Faure, G. and Rougerie, F. 1996. Deglacial sea-level record from Tahiti corals and the timing of global meltwater discharge, Nature, 382, 241-244.
- ↑ Weaver, A.J., Saenko, O.A., Clark, P.U. and Mitrovica, J.X. 2003. Meltwater Pulse 1A from Antarctica as a trigger of the Bølling-Allerød warm interval, Science, 299, 1709-1713.
- ↑ Peltier, W.R. 2005. On the hemispheric origins of meltwater pulse 1a, Quaternary Science Reviews, 24, 1655-1671.
- ↑ 13.0 13.1 13.2 Verleyen, E., Hodgson, D.A., Milne, G.A., Sabbe, K. and Vyverman, W. 2005. Relative sea level history from the Lambert Glacier region (East Antarctica) and its relation to deglaciation and Holocene glacier re-advance, Quaternary Research, 63, 45-52.
- ↑ 14.0 14.1 14.2 Bassett, S.E., Milne, G.A., Bentley, M.J. and Huybrechts, P. 2007. Modelling Antarctic sea-level data to explore the possibility of a dominant Antarctic contribution to meltwater pulse IA, Quaternary Science Reviews, 26, 2113-2127.
- ↑ Baroni, C. and Orombelli, G. 1994. Abandoned pengiun rookeries as Holocene paleoclimatic indicators in Antarctica, Geology, 22, 23-26.
- ↑ 16.0 16.1 16.2 Hall, B.L., Baroni, C. and Denton, G.H. 2003. Holocene relative sea-level history of the southern Victoria Land coast, Antarctica, Global and Planetary Change, 42, 241-263.
- ↑ 17.0 17.1 Bentley, M.J., Hodgson, D.A., Smith, J.A. and Cox, N.J. 2005a. Relative sea level curves for the South Shetland Islands and Marguerite Bay, Antarctic Peninsula, Quaternary Science Reviews, 24, 1203-1216.
- ↑ 18.0 18.1 Conway, H., Hall, B.L., Denton, G.H., Gades, A.M., Waddington, E.D. 1999. Past and future grounding-line retreat of the West Antarctic Ice Sheet. Science 286:280-283.
- ↑ Zwartz, D., Bird, M., Stone, J. and Lambeck, K. 1998. Holocene sea-level change and ice-sheet history in the Vestfold Hills, East Antarctica, Earth and Planetary Science Letters, 155, 131-145.
- ↑ Ó Cofaigh, C., Pudsey, C.J., Dowdeswell, J.A. and Morris, P. 2002. Evolution of subglacial bedforms along a paleo-ice stream, Antarctic Peninsula continental shelf, Geophysical Research Letters, 29, 1199.
- ↑ Nakada, M., Kimura, R., Okuno, J., Moriwaki, K., Miura, H. and Maemoku, H. 2000. Late Pleistocene and Holocene melting history of the Antarctic ice sheet derived from sea-level variations, Marine Geology, 167, 85-103.
- ↑ Ivins, E.R. and James, T.S. 2005. Antarctic glacial isostatic adjustment: a new assessment, Antarctic Science, 17, 541-553.