Small-scale processes in the Southern Ocean
- This page is part of the topic The Southern Ocean in the instrumental period
The importance of small-scale processes in surface, bottom and lateral boundary layers is well known. In ice-covered oceans the surface boundary layer is of particular interest (McPhee, 2009) since it controls the heat and momentum exchange between ice and ocean. These exchanges affect ice growth, melt and motion as well as the heat budget of the ocean and the currents (McPhee et al., 2008[1]). Appropriate quantification of such processes is a prerequisite for successful modelling the large scale conditions.
For a long time small-scale processes related to ocean turbulence in the interior were almost neglected in the consideration of large-scale ocean conditions. This resulted from lacking appropriate observation techniques and theoretical concepts for relating small-scale to large-scale processes. Normally a simple energy cascade providing turbulent energy from larger scale ocean current shear to small-scale mixing was assumed, which could be applied in large-scale models by properly selected mixing parameters. However, improved measurements of turbulence suggest that the amount of turbulent energy is much larger in the interior than previously assumed. There, internal waves, not only generated at the continental slope, but as well over rough bottom topography, play a major role in generating intensive mixing in the interior away from the well-known strongly mixed boundary layers. The intensity of internal mixing is so high, that it has to be taken into account to quantitatively understand the meridional overturning circulation. The understanding of spatially and timely important variations in the intensity of mixing and its significant role for large-scale processes prompted research with the aim of detecting by observations whether changes in small-scale processes could affect large-scale conditions i.e. the oceans role in climate.
It has been estimated (e.g., Wunsch, 1998[2]) that up to one-third of the energy required to drive the global ocean’s overturning circulation (2-3 TW, see Wunsch and Ferrari (2004[3]) for a review) stems from the work done by the wind on the ACC, and that the bulk of that energy is transferred to the mesoscale eddy field. Current conceptual and numerical models of the Southern Ocean overturning circulation (see Rintoul et al. (2001[4]) and Olbers et al. (2004[5]) for two reviews) unanimously highlight the eddies’ crucial role in transporting water masses and tracers along the sloping isopycnals (surfaces of constant density) of the ACC — particularly in the upper overturning cell — as well as in transporting the momentum input by the wind to the level of topographic obstacles, such as ridges, where it may be transferred to the solid Earth.
Physical processes occurring on length scales on which earth rotation is of similar importance to ocean stratification (the baroclinic Rossby radius of deformation), which are smaller than 5-20 km south of 40ºS, (see Chelton et al., 1998[6]) play an important role in shaping and driving the circulation of the Southern Ocean. To represent ocean properties realistically measurements and models would need to resolve this scale. However this is normally not possible and the lack of resolution has to be compensated for by assumptions about processes at this scale and below.
For example, formation of the AABW filling the deepest layers of much of the global ocean abyss is crucially dependent on the convective (i.e. small scale) production of dense, high-salinity shelf waters over the Antarctic continental shelves during periods of sea ice growth (Morales Maqueda et al., 2004[7]), as well as on the heat and freshwater exchanges between those waters and the adjacent ice shelves (e.g. Hellmer, 2004[8]). The properties of Antarctic shelf waters are modified further by turbulent mixing associated with the dissipation of tidal energy taking place over the continental shelves fringing the continent (Egbert and Ray, 2003[9]), including the vicinity of the ice shelf front (Makinson et al., 2006[10]) and sub-ice-shelf cavities (Makinson, 2002[11]). A variety of small-scale processes underlies the conversion of tidal energy into turbulence, most prominent are the breaking of internal gravity waves generated by tidal flows impinging on rough ocean-floor topography (e.g., Robertson et al., 2003[12]), and the formation of thick frictional boundary layers (e.g., Makinson, 2002[11]). The importance of these processes is accentuated by the proximity of the Antarctic continental shelves to the critical latitude (where the tidal frequency is equal to the one of inertial oscillations imposed on ocean flow by the Earth’s rotation) of the dominant tidal constituent (M2), near which the generation of internal tides and frictional boundary layers is most efficient (Robertson, 2001[13]; Pereira et al., 2002[14]). In addition to their role in moulding the properties of shelf waters, tidal fluctuations regulate the flow of those waters across the ice shelf front (Nicholls et al., 2004[15]) and the continental shelf break (e.g. Gordon et al., 2004[16]), often steered by local topographic features such as canyons. On descending the continental slope in broad sheets or narrow plumes, shelf waters tend to focus in largely geostrophic boundary currents that entrain ambient surface and intermediate waters and detrain ventilated fluid in the offshore direction (Baines and Condie, 1998[17]; Hughes and Griffiths, 2006[18]). The shelf waters’ descent is promoted further by other smaller scale processes, such as double-diffusion (instabilities due to different diffusivities of heat and salt) and interleaving (layer formation) across the shelf break zone (Foster and Carmack, 1976[19]; Foster, 1987[20]), as well as nonlinearities in the equation of state (thermobaricity and cabbeling) and instabilities of the Antarctic Slope Front, which have been reported to generate cyclonic eddies effecting a net downward transport of shelf water (see Baines and Condie, 1998[17] for a review). In subsequent stages of its northward journey, the newly formed AABW navigates numerous topographic obstacles and, in so doing, undergoes profound modification due to vigorous turbulent mixing with overlying water masses (e.g. Heywood et al., 2002[21]). A large fraction of this modification is likely driven by flows over small sills in confined passages (Bryden and Nurser, 2003[22]) and mid-ocean ridge-flank canyons (Thurnherr and Speer, 2003[23]), although the breaking of internal tides (Simmons et al., 2004[24]) and internal lee waves (Naveira Garabato et al., 2004[25]) must contribute significantly too.
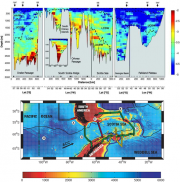
While theoretical considerations (Marshall and Naveira Garabato, 2007) and altimetric observations of an energy cascade from smaller to larger motions in the Southern Ocean (Scott and Wang, 2005[26]) endorse the view that much of the eddy field’s energy is ultimately transferred toward the ocean floor and dissipated by viscous bottom drag, measurements of oceanic fine structure (Naveira Garabato et al., 2004[25]; Sloyan, 2005[27]; Kunze et al., 2006[28]) suggest that a substantial fraction of the energy is dissipated via the generation and breaking of internal lee waves. These waves induce intense turbulent mixing (Figure 4.28) and, in so doing, contribute to driving the lower cell of the Southern Ocean overturning. It thus appears that the upper and lower overturning cells, often treated as largely independent entities in descriptions of the ocean circulation, may be strongly coupled by smaller scale processes. This proposition is consistent with the concurrent intensification of eddy-driven upwelling along inclined surfaces of constant water density, and turbulent mixing of superimposed water layers of different density (diapycnal mixing) in ACC regions of complex topography. In this view, eddy dampening - which is required to sustain a vigorous meridional circulation in the upper ocean - may be connected to the topographic generation of internal lee waves in the abyss (Naveira Garabato et al., 2007[29]). Although the patchy indirect evidence available to date points to topographic generation as the key agent in the transfer of eddy energy to the internal wave field in the ACC (Figure 4.28), other mechanisms are likely to enhance this transfer e.g. interaction between internal waves and mesoscale eddies (Polzin, 2007[30]) and the generation of internal waves by unstable mesoscale processes (Molemaker et al., 2005[31]). The occurrence of these processes is indicated by altimetric evidence of an energy cascade at rather small scales in the Pacific sector of the ACC (Scott and Wang, 2005[26]). Nonetheless, it appears that diapycnal mixing in these upper layers of the ACC is primarily driven by the breaking of downward-travelling near-inertial internal waves generated by the wind in the upper-ocean mixed layer (Alford, 2003[32]).
The key point emerging is that relatively small scale processes in the Southern Ocean exert an important influence on the large-scale behaviour of global ocean circulation over a wide range of time scales of climatic significance. This is a highly significant conclusion when one considers that these processes and interactions are often absent, or parameterized with coefficients tuned to the present ocean state, in the models used to simulate the ocean’s evolution (see e.g. Wunsch and Ferrari (2004[3]) for a discussion).
References
- ↑ McPhee, M.G., Morison, J.H. and Nilsen, F. 2008. Revisiting heat and salt exchange at the ice-ocean interface: Ocean flux and modelling considerations, Journal of Geophysical Research, 113, C06014, doi: 10.1029/2007JC004383.
- ↑ Wunsch, C. 1998. The work done by the wind on the oceanic general circulation, J. Phys. Oceanogr, 28, 2332-2340.
- ↑ 3.0 3.1 Wunsch, C. and Ferrari, R. 2004. Vertical mixing, energy, and the general circulation of the oceans, Ann. Rev. Fluid Mech., 36, 281-314.
- ↑ Rintoul, S.R., Hughes, C.W. and Olbers, D. 2001. The Antarctic Circumpolar Current system. In: Eds. G. Siedler, J. Church and J. Gould, Ocean circulation and climate; observing and modelling the global ocean. International Geophysics Series, 77, 271-302, Academic Press.
- ↑ Olbers, D., Borowski, D., Völker, C. and Wolff, J.-O. 2004. The dynamical balance, transport and circulation of the Antarctic Circumpolar Current, Antarct. Sci., 16, 439-470.
- ↑ Chelton, D.B., Deszoeke, R.A., Schlax, M.G., EL Naggar, K. and Siwertz, N. 1998. Geographical variability of the first-baroclinic Rossby radius of deformation, J. Phys. Oceanogr., 28, 433-460.
- ↑ Morales Maqueda, M.A., Willmott, A.J. and Biggs, N.R.T. 2004. Polynya dynamics: A review of observations and modelling, Rev. Geophys., 42, doi:10.1029/2002RG000116.
- ↑ Hellmer, H.H. 2004. Impact of Antarctic shelf basal melting on sea ice and deep ocean properties, Geophys. Res. Lett., 31, doi:10.1029/2004GL019506.
- ↑ Egbert, G.D. and Ray, R.D. 2003. Semi-diurnal and diurnal tidal dissipation from TOPEX/Poseidon altimetry, Geophys. Res. Lett., 30, doi:10.1029/2003GL017676.
- ↑ Makinson, K., Schröder, M. and Osterhus, S. 2006. Effect of critical latitude and seasonal stratification on tidal current profiles along Ronne Ice Front, Antarctica, J. Geophys. Res., 111, doi:10.1029/2005JC003062.
- ↑ 11.0 11.1 Makinson, K. 2002. Modeling tidal current profiles and vertical mixing beneath Filchner-Ronne Ice Shelf, Antarctica, J. Phys. Oceanogr., 32, 202-215.
- ↑ Robertson, R., Beckmann, A. and Hellmer, H. 2003. M2 tidal dynamics in the Ross Sea, Antarct. Sci., 15, 41-46.
- ↑ Robertson, R. 2001. Internal tides and baroclinicity in the southern Weddell Sea: Part II: Effects of the critical latitude and stratification, J. Geophys. Res., 106, 27017-27034.
- ↑ Pereira, A.F., Beckmann, A. and Hellmer, H.H. 2002. Tidal mixing in the southern Weddell Sea: Results from a three-dimensional model, J. Phys. Oceanogr., 32, 2151-2170.
- ↑ Nicholls, K.W., Makinson, K. and Osterhus, S. 2004. Circulation and water masses beneath the northern Ronne Ice Shelf, Antarctica, J. Geophys. Res., 109, doi:10.1029/2004JC002302.
- ↑ Gordon, A. L., Zambianchi, E., Orsi, A., Visbeck, M., Giulivi, C. F., Whitworth III, T. and Spezie, G. 2004. Energetic plumes over the western Ross Sea continental slope, Geophys. Res. Lett., 31, doi: 10.1029/2004GL020785.
- ↑ 17.0 17.1 Baines, P.G. and Condie, S. 1998. Observations and modelling of Antarctic downslope flows: a review. In Ocean, Ice and Atmosphere: Interactions at the Antarctic Continental Margin, AGU Antarctic Research Series Vol. 75, S.S. Jacobs and R. Weiss editors, 29-49.
- ↑ Hughes, G.O. and Griffiths, R.W. 2006. A simple convective model of the global overturning circulation, including effects of entrainment into sinking regions, Ocean Model., 12, 46-79.
- ↑ Foster, T.D. and Carmack, E.C. 1976. Temperature and salinity structure in the Weddell Sea, J. Phys. Oceanogr., 6, 36-44.
- ↑ Foster, T.D. 1987. Mixing and bottom water formation in the shelf break region of the southern Weddell Sea, Deep-Sea Res. 34, 1771-1794.
- ↑ Heywood, K.J., Naveira Garabato, A.C. and Stevens, D.P. 2002. High mixing rates in the abyssal Southern Ocean, Nature, 415, 1011-1014.
- ↑ Bryden, H. L. and Nurser, A.J.G. 2003. Effects of strait mixing on ocean stratification, J. Phys. Oceanogr., 33, 1870-1872.
- ↑ Thurnherr, A.M. and Speer, K.G. 2003. Boundary mixing and topographic blocking on the Mid-Atlantic Ridge in the South Atlantic, J. Phys. Oceanogr., 33, 848-862.
- ↑ Simmons, H., Hallberg, R. and Arbic, B. 2004. Internal wave generation in a global baroclinic tide model, Deep-Sea Res. II, 51, 3043-3068.
- ↑ 25.0 25.1 25.2 Naveira Garabato, A.C., Polzin, K.L., King, B.A., Heywood, K.J. and Visbeck, M. 2004. Widespread intense turbulent mixing in the Southern Ocean, Science, 303, 210-213.
- ↑ 26.0 26.1 Scott, R.B. and Wang, F. 2005. Direct evidence of an oceanic inverse kinetic energy cascade from satellite altimetry, J. Phys. Oceanogr., 35, 1650-1666.
- ↑ Sloyan, B.M. 2005. Spatial variability of mixing in the Southern Ocean, Geophys. Res. Lett., 32, doi:10.1029/2005GL023568.
- ↑ Kunze, E., Firing, E., Hummon, J.M., Chereskin, T.K. and Thurnherr, A.M. 2006. Global abyssal mixing inferred from lowered ADCP shear and CTD strain profiles, J. Phys. Oceanogr., 36, 1553-1576.
- ↑ Naveira Garabato, A.C., Stevens, D.P., Watson, A.J. and Roether, W. 2007. Short-circuiting of the overturning circulation in the Antarctic Circumpolar Current, Nature, 447, 194-197.
- ↑ Polzin, K.L. 2007. How Rossby Waves Break. Results from POLYMODE and the end of the enstrophy cascade, J. Phys. Oceanogr., in press.
- ↑ Molemaker, J., McWilliams, J.C. and Yavneh, I. 2005. Baroclinic instability and loss of balance, J. Phys. Oceanogr. 35, 1505-1517.
- ↑ Alford, M.H. 2003. Improved global maps and 54-year history of wind-work on ocean inertial motions. Geophys. Res. Lett., 30, doi:10.1029/2002GL016614.