Atmospheric circulation changes in the instrumental period
- This page is part of the topic Antarctic climate and environment change in the instrumental period
Modes of variability
Variability
Within the atmospheric circulation of the high southern latitudes several so-called modes of variability can be discriminated. These circulation patterns appear more frequently than would be expected in a random sample, and can ‘describe’ a significant proportion of the total circulation variability.
The Southern Annular Mode
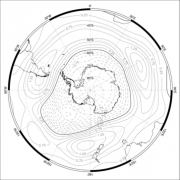
As pointed out in The role of the Antarctic in the global climate system, the Southern Annular Mode (SAM) is the principal mode of the Southern Hemisphere extra-tropical atmospheric circulation. It can be described either as a ‘flip-flop’ of atmospheric mass between mid- and high-latitudes, such that there are synchronous pressure (or geopotential height) anomalies of opposite sign in these two regions (e.g. Rogers and van Loon, 1982[1]), or as a north-south shift in the mid-latitude jet resulting from both latitudinal vacillations in the jet and fluctuations in jet strength (Fyfe and Lorenz, 2005[2]). When pressures are higher (lower) than average over the Southern Hemisphere mid-latitudes (Antarctica) the SAM is said to be in its positive phase (see Figure 4.1) and vice versa.
The spatial pattern of the SAM varies negligibly with height in the atmosphere and is revealed as the leading mode of variability in many atmospheric fields (see Thompson and Wallace (2000[3]) and references therein). Model experiments demonstrate that the structure and variability of the SAM results from the internal dynamics of the atmosphere (e.g. Limpasuvan and Hartmann, 2000[4]). Synoptic-scale (a horizontal scale of greater than 1,000 km) weather systems interact with the zonal mean flow to sustain latitudinal displacements of the mid-latitude westerlies. The SAM contributes a significant proportion of Southern Hemisphere climate variability (typically ~35%) from high-frequency to very low-frequency timescales, with this variability displaying a greater tendency to low frequency variability.
Gridded reanalysis datasets have been used to derive time series of the SAM (e.g. Renwick, 2004). Due to the poor quality of current reanalyses products at high southern latitudes prior to the assimilation of satellite sounder data in the late 1970s (Hines et al., 2000[5]), long-term SAM time-series cannot be derived from them. Based on a definition by Gong and Wang (1999[6]), Marshall (2003[7]) produced a SAM index based on 12 appropriately located station observations in the extra-tropics and coastal Antarctica. This index itself is limited to the period following the IGY. Attempts have been made to reconstruct annual to century-scale records based on the SAM and associated atmospheric circulation features, such as surface pressure over Antarctica and the Southern Hemisphere westerlies. Souney et al. (2002[8]) used variability in Na concentrations from the Law Dome ice core, while Jones and Widmann (2003[9]) employed tree-ring width chronologies: both these studies highlight the decadal variability in their derived SAM time series. Yan et al. (2005[10]) reconstructed past behaviour of the Southern Hemisphere westerlies at the edge of the polar vortex using the variability in Ca concentrations from West Antarctic ice cores.
The SAM has shown significant positive trends over the past few decades, particularly during austral autumn and summer (e.g. Thompson et al., 2000[12]; Marshall, 2003[7]). The positive trend was especially pronounced from the mid-1960s until the end of the Twentieth Century, since when there has been a similar frequency of seasons with positive and negative SAM values (Figure 4.2). The positive trend in the SAM has resulted in a strengthening of the mean circumpolar westerlies of ~15% (Marshall, 2002[13]), and contributed to the spatial variability in Antarctic temperature change (e.g. Thompson and Solomon, 2002[14]; Kwok and Comiso, 2002[15]; Schneider et al., 2004[16]; Marshall, 2007[17]), specifically a warming in the northern Peninsula region and a cooling over much of the rest of the continent. The SAM also impacts the spatial patterns of variability in precipitation across Antarctica (e.g. Genthon et al., 2003[18]).
The imprint of SAM variability on the Southern Ocean system is observed as a coherent sea level response around Antarctica (Aoki, 2002[19]; Hughes et al., 2003[20]), and by its regulation of Antarctic Circumpolar Current (ACC) flow through the Drake Passage (Meredith et al., 2004[21]). Modelling studies show that a positive phase of the SAM is associated with northward (southward) Ekman drift in the Southern Ocean (at 30(S) leading to upwelling (downwelling) near the Antarctic continent (~45SS) (Hall and Visbeck, 2002[22]; Lefebvre et al., 2004[23]). These changes in oceanic circulation impact directly on the thermohaline circulation and may explain recent patterns of observed oceanic temperature change in the Southern Ocean described by Gille (2002[24]). Although the SAM is essentially zonal, a wave-number 3 pattern (three troughs and three ridges around the hemisphere) is superimposed, with a marked low pressure anomaly west of the Peninsula when the SAM is positive, leading to increased northerly flow and reduced sea ice in the region (Liu et al., 2004[25]). Raphael (2003[26]) reported that diminished summer sea ice may in turn feed back into a more positive SAM. However, modelling work by Marshall and Connolley (2006[27]) showed that increased sea surface temperatures (SSTs) at high southern latitudes will warm the atmosphere, and, through thermodynamical processes, cause the atmospheric centre-of-mass to rise and geopotential height to increase thus producing a more negative SAM.
The Pacific-South American (PSA) pattern
The El Niño–Southern Oscillation (ENSO) phenomenon is the largest climatic cycle on Earth on decadal and sub-decadal time scales, and can influence the weather and climate well beyond the low-latitude Pacific Ocean where it is most marked. ENSO is a coupled atmosphere-ocean phenomenon that involves a major reversal of the atmospheric and oceanic flows across the tropical Pacific Ocean. During the La Niña phase there is intense storm activity close to Indonesia and strong westward moving atmospheric and ocean flow across the Pacific near the Equator. During the El Niño phase the storm activity moves close to the date-line, with deep convection giving upper atmosphere divergence. This results in a quasi-stationary Rossby Wave (atmospheric long wave) pattern becoming established in both hemispheres, so providing teleconnection patterns between high and low latitudes.
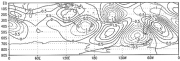
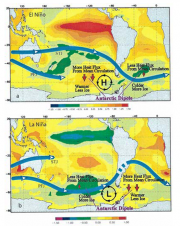
In the Southern Hemisphere, the Rossby wave train is known as the Pacific-South American Association (PSA) and it links the central tropical Pacific and the Amundsen-Bellingshausen Sea (ABS) (Figure 4.3). The most robust signals of ENSO are found across the South Pacific during winter and at this time of year, the wave train can be identified as anomalously high mean sea level pressure (MSLP) and upper level heights (heights of constant pressure surfaces) across the ABS, with anomalously low values to the east of New Zealand (Figure 4.4) (Karoly, 1989[30]). Such circulation anomalies give generally colder temperatures across the Antarctic Peninsula, with more extensive sea ice, and warmer air arriving from the north across West Antarctica and the Ross Ice Shelf (Figure 4.4).
During the La Niña phase of the cycle these anomaly patterns are broadly reversed with more cyclonic activity over the ABS and warmer winters experienced over the Antarctic Peninsula (Turner, 2004[31]) (Figure 4.4).
The PSA pattern is thought to be primarily related to ENSO. Nonetheless, it is prominent at many timescales even in the absence of a strong ENSO signal. Thus, the PSA may also be an internal mode of climate variability. Note that Mo and Higgins (1998[28]) state that the PSA actually comprises two separate modes, each associated with enhanced convection in different parts of the Pacific and suppressed convection elsewhere.
An alternative mechanism for the PSA teleconnection was proposed by Liu et al. (2002[32]). They suggest that the increased convection associated with El Niño events alters the mean meridional atmospheric circulation through longitudinal changes to the Hadley circulation and subsequent alterations of the subtropical jet position and strength. Yuan (2004[29]) suggests that the two mechanisms operate in phase and are comparable in magnitude.
While there are general patterns of high-latitude climate anomalies that co-vary with ENSO, correlations suggest that teleconnections are small. A modelling study by Lachlan-Cope and Connolley (2006[33]) shows that this is because (i) Rossby wave dynamics are not well-correlated to ‘standard’ definitions of ENSO, because the relationship between upper level divergence and SSTs via deep convection is complex, and (ii) natural variation in the zonal flow of Southern Hemisphere high-latitudes can swamp the ENSO signal. Moreover, Fogt and Bromwich (2006[34]) demonstrated that a weaker high-latitude teleconnection in spring during the 1980s compared to the following decade was due to the out-of-phase relationship between the PSA and SAM at this time: subsequently, the tropical and extra-tropical modes of climate variability had an in-phase relationship.
Ice core records have provided insight into the tropical-Antarctic teleconnections in the pre-instrumental period. Schneider and Steig (2008[35]) found positive temperature anomalies in West Antarctica over the period 1936-45, which they attributed to the major 1939-42 El Niño event.
Although apparent throughout the year, the PSA demonstrates the strongest teleconnections to the Antarctic region in austral spring and summer. During these seasons an El Niño (or a La Niña) event is associated with significantly more (or less) blocking events in the southeast Pacific (Renwick and Revell, 1999[36]). This pressure anomaly in the ABS is primarily responsible for the Antarctic Dipole (ADP) in the interannual variance structure in the sea ice edge and SST fields of the Southern Ocean, which are characterised by an out-of-phase relationship between anomalies in the central/eastern Pacific (ABS) and the Atlantic (Weddell Sea) sectors (Yuan and Martinson, 2001[37]: see Figure 4.4). In addition, the ADP sea ice anomalies are reinforced by ENSO-related storm track variability, which influences the Ferrel cell (the vertical circulation cell located between the Hadley Cell of the tropics and the Polar Cell of the high latitude areas) by changing the meridional heat flux divergence/convergence and shifting the latent heat release zone, which in turn modulates the mean meridional heat flux that impacts sea ice extent. Correlations with ENSO indices imply that up to 34% of the variance in sea ice edge is linearly related to ENSO (Yuan and Martinson, 2000[38]), while Gloersen (1995[39]) showed that different regions of sea ice respond to ENSO at different periodicities. The strongest sea ice correlations associated with ENSO occur at 120-132º W (in the ABS) lagging the tropical temperature anomaly by 6 months; so the austral spring/summer ENSO signal is observed in the subsequent ice growth period in autumn/winter (Yuan and Martinson, 2000[38]). Note that the temporal quasi-periodic nature of both ENSO and the ice anomalies prevents the identification of direction of causality. Kwok and Comiso (2002[15]) state that recent trends in sea ice extent in both the Bellingshausen Sea and Ross Sea are related directly to ENSO variability.
The Antarctic Circumpolar Wave
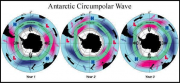
The Antarctic Circumpolar Wave (ACW), first defined by White and Peterson (1996[40]), is an apparent easterly progression of phase-locked anomalies in Southern Ocean surface pressure, winds, SSTs and sea-ice extent (Figure 4.5). It thus represents a coupled mode of the ocean-atmosphere system. The ACW has a zonal wavenumber of 2 (meaning a wavelength of 180°), and the anomalies propagate at a speed of 6-8 cm/sec such that they take 8-10 years to circle Antarctica, giving the ACW a period of 4-5 years. A similar feature has also been identified in sea surface height using satellite altimeter data (Jacobs and Mitchell, 1996[41]). Given the much shorter response times of the atmosphere, these authors proposed that the ocean plays an important part in creating and maintaining the ACW. As there is little Antarctic multiyear ice, Gloersen and White (2001[42]) suggested that the memory of the ACW in the sea ice pack is carried from one austral winter to the next by the neighbouring SSTs. White et al. (2004[43]) showed a complex tropospheric response to sea ice anomalies that, for example, explained anomalous poleward surface winds and deep convection observed with negative sea ice edge anomalies.
Some authors have suggested that an ACW signal can be observed in an Antarctic ice core over the last 2000 years (Fischer et al., 2004[44]). But, since the initial discovery of the ACW, others have questioned its persistence. Several observational and modelling studies have indicated that the ACW is not apparent in recent data before 1985 and after 1994 (e.g. Connolley, 2003[45]), which is somewhat fortuitously the period that White and Peterson (1996[40]) chose for their original analysis. In addition, there has been some discussion on one of its key characteristics, whether it really has a wave number 2 pattern. Many studies (e.g. Cai et al. 1999[46]) have indicated that an ACW-like feature is apparent in GCM control runs but that it has a preferred wavenumber 3 pattern. Venegas (2003[47]), using frequency domain decomposition, suggested that the ACW comprises two significant interannual signals that combine constructively/destructively to give the observed irregular fluctuations of ACW on interannual time scales, as also seen in GCM studies. The two signals comprise (i) a 3.3 year period of zonal wavenumber 3 and (ii) a 5 year period of zonal wavenumber 2, which was particularly pronounced during the period studied by White and Peterson.
However, most of the ACW debate has centred on its forcing mechanisms and, as a consequence, the very nature of its existence. For example, White et al. (2002[48]) state explicitly that the ACW exists independently of the tropical standing mode of ENSO, and that its eastward propagation depends upon atmosphere-ocean coupling rather than on advection by the ACC: both these points have been refuted in the literature. The ADP (Yuan and Martinson, 2001[37]), described previously, has the same wavelength as the ACW, but key differences are that the associated variability is twice that of the ACW, and that the dipole consists principally of a strong standing mode together with a much weaker propagating motion. Moreover, Yuan and Martinson (2001[37]) state that the ADP is clearly associated with ENSO events. Several other authors have found that ENSO variability is important in driving a geographically fixed standing wave in Southern Ocean interannual variability, that is centred in the South Pacific and linked to the PSA pattern (Cai et al., 1999[46]; Venegas, 2003[47]; Park Y. et al., 2004).
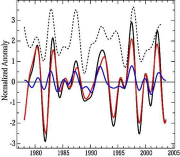
Furthermore, several papers mention the ACC as the means of anomaly propagation. In an ocean model forced by realistic stochastic (random) anomalies, Weisse et al. (1999[49]) showed that the ocean acts as an integrator of short-term atmospheric fluctuations (white noise) and turns them into a lower frequency signal (red noise); subsequently the average zonal velocity of the ACC determines the timescale of the oceanic variability and thus the propagation speed and period of the ACW (Cai et al., 1999[46]; Venegas, 2003[47]). The results of the study of Park Y. et al. (2004) indicated that any such propagating anomalies comprised only ~25% of interannual Southern Ocean SST variability and are often rapidly dissipated in the Indian Ocean, are intermittent in phase and frequently do not complete a circumpolar journey (Figure 4.6). Hence, they questioned the existence of the ACW, as originally described by White and Peterson (1996[40]).
Depression Tracks
The paths of cyclones, known as depression or storm tracks, can be obtained using three broad methodologies. First, individual weather systems can be identified by their cloud signatures in satellite imagery or meteorological charts, and their subsequent paths can then be determined from further multitemporal imagery. The polar regions are well-suited to this kind of analysis because polar orbiting satellites provide many overlapping passes at high latitudes. Although attempts have been made to automate this process, most studies to date have been done manually, and the labour-intensive nature of this process means that the time periods covered are relatively short. For example, Turner et al. (1998[50]) examined 12 months of AVHRR data in the Antarctic Peninsula sector during which 504 synoptic-scale lows were identified.
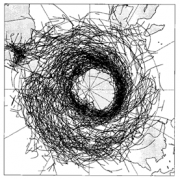
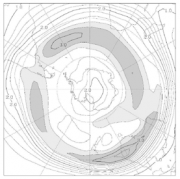
The availability of gridded data from numerical weather prediction (NWP) models and reanalyses allows entirely automated methods to be used that can provide climatological information on depression tracks, including trends. System-centred tracking is achieved by searching for local minima in certain fields, such as MSLP (e.g. Jones and Simmonds, 1993[51]), or maxima in vorticity (e.g. Hoskins and Hodges, 2005[52]). An example of the resultant tracks at high southern latitudes is shown in Figure 4.7a. A final methodology is to utilise Eulerian storm-track diagnostics by identifying the variance of vorticity at synoptic-timescales (2-6 days). Figure 4.7b is an equivalent to figure 4.7a using that method: note the significant difference in results in the circumpolar trough (CPT) with the system-centred approach finding a maximum, while the Eulerian method indicates relatively little cyclone activity at that site. The satellite-imagery study of Turner et al. (1998[50]) reveals that there is indeed a maximum in cyclone activity at that site.
There is a marked difference between the main CPT storm track in austral summer and winter. In the summer it is nearly circular and confined to high-latitudes south of 50°S. In winter (Fig 4.7a) the storm track is more asymmetric with a spiral from the Atlantic and Indian Oceans in towards Antarctica, and a pronounced sub-tropical jet (STJ) - related storm track at ~35°S over the Pacific (e.g. Hoskins and Hodges, 2005[52]). Simmonds and Keay (2000[53]) found that the mean track length of winter systems (2,315 km) was slightly longer than in summer (1,946 km). The equinoctial seasons have depression track patterns intermediate between summer and winter. The CPT has a maximum in storm activity in the Atlantic and Indian Ocean regions at all times of year. While the previous description relates to the mean climatology, individual weather systems can behave differently; for example, many studies have shown cases where cyclones have a strong northerly track, particularly when associated with cold-air outbreaks from the Antarctic continent. The automated procedures can also be used for locating and tracking anticyclones. In such a study, Sinclair (1996[54]) found that south of 50°S anticyclones were generally rare, but their tracks were associated with the main regions of blocking in the South Pacific as described previously.
Using gridded data, Simmonds and Keay (2000[53]) showed that annual and seasonal numbers of cyclones have decreased at most locations south of 40°S during the 1958-97 period examined, and can be related to changes in the SAM. The latter is associated with a decline in pressure around Antarctica so there has been a trend to fewer but more intense cyclones in the circumpolar trough. One exception is the Amundsen-Bellingshausen Sea region where cyclonic activity has increased (Simmonds et al., 2003[55])
Depression formation and decline
Depression tracking studies can also show the locations where a weather system is first and last identified, respectively cyclogenesis and cyclolysis. By collating data from all cyclones examined, regions of preferred cyclogenesis and cyclolysis may be determined. Using data from the NCEP-NCAR reanalysis, Simmonds and Keay (2000[53]) showed there to be a net creation of cyclones (i.e. cyclogenesis is greater than cyclolysis) north of 50°S and net destruction south of this. Most Southern Hemisphere cyclogenesis actually occurs at very high latitudes (~60°S) in the CPT but rates of cyclolysis there are even higher. Turner et al. (1998[50]) found that about half the systems in the Antarctic Peninsula region formed within the CPT and half spiralled in from further north. Simmonds et al. (2003[55]) also revealed the northern part of the Peninsula to be a region of high cyclogenesis. Some of these systems formed through lee cyclogenesis — a dynamical process associated with the passage of air over a barrier — to the east of the Antarctic Peninsula. Other important regions of cyclogenesis within the CPT are in the Indian Ocean sector, with a maximum at 65°S, 150°E (Hoskins and Hodges, 2005[52]) at the edge of the sea ice. Cyclolysis is generally confined to the CPT, with maxima in the Indian Ocean and also the Bellingshausen Sea, where the steep, high Antarctic Peninsula often prevents weather systems passing further to the east: such areas are known as ‘cyclone graveyards’.
Blocking events
Normally, low pressure systems do not intrude into the high inland area. Sometimes, in winter, blocking events occur and depressions from lower latitudes are channelled south over the ice sheet (Enomoto et al., 1998[56]; Hirasawa et al., 2000[57]; Pook and Gibson, 1999[58]; Schneider et al., 2004[16]). During these blocking events, an abrupt increase of surface temperature up to 40ºC may occur within a few days, accompanied by a heavy snowfall, that significantly influences accumulation on the inland ice sheet. These events are not common, but may have a pronounced effect on the energy balance of inland areas and on the annual layer formation seen in ice cores.
Teleconnections
Atmospheric linkages
The Pacific-South American (PSA) pattern above describes the teleconnections between the ENSO cycle of the tropical Pacific and the Antarctic. However, a problem is that while many of the El Niño and La Niña events give the atmospheric anomaly patterns described earlier, some of these events have resulted in very different conditions. For example, the 1982/83 El Niño event was one of the largest of the last century, but the region of positive MSLP/height anomaly was displaced towards the tip of South America so the Antarctic Peninsula had only average conditions rather than the low temperatures usually associated with El Niño events. In fact, the PSA is generally more variable than the Pacific North American Association, making it much more difficult to anticipate how the Antarctic atmosphere will respond as an El Niño event starts to become established.
In recent decades there has been a trend towards more frequent and more intense El Niño events. We could therefore expect MSLP values to have risen across the ABS and there to have been colder winter season temperatures across the Antarctic Peninsula and warmer conditions in the Ross Sea area. However, this has not happened, indicating that the winter warming on the western side of the Peninsula is not a direct result of changes in ENSO.
Bertler et al. (2004[59]) noted a shift of the Amundsen Sea Low eastwards during some El Niño events and such a result would be consistent with the recent observed cooling in the Ross Sea area (Doran et al., 2002[60]). However, this area has experienced jumps in the relationship between ENSO and West Antarctic precipitation (Cullather et al., 1996[61]) indicating the variable nature of the teleconnections. In addition, the Antarctic Peninsula, far from cooling, has experienced the largest increase of temperature anywhere in the Southern Hemisphere (Turner et al., 2005a[62]).
In summary, while the relatively short timeseries that we have of Antarctic meteorological observations and atmospheric analyses do suggest that tropical atmospheric and oceanic conditions affect the climate of the Antarctic and the Southern Ocean the connections do vary with time and are rather non-linear. Ice core proxies for El Niño are emerging to help fll this gap, notably the MSA proxy for the last 500 years of El Niño produced from a South Pole ice core (Meyerson et al., 2002[63]). However, the teleconnections are not as robust as those in the Northern Hemisphere. In addition, many other factors in the Antarctic climate system, such as the variability in the ocean circulation, the development of the ozone hole and the large natural variability of the high latitude climate, all affect atmospheric conditions and can mask the tropical signals.
Oceanic Coupling
The atmospheric processes described above have strong impacts on the high latitude Southern Ocean, which can (via complex feedback mechanisms) produce changes to the large-scale coupled atmosphere/ocean/ice system. The atmospheric Rossby wave associated with ENSO (the PSA association) produces anomalies in SSTs across the Southern Ocean, via processes including changes to the surface heat budget associated with changes in clouds and radiation (Li, 2000[64]). These changes are especially manifest in the South Pacific (Figure 4.4).
The SST anomalies so created lie largely within the domain of the ACC, and hence are subsequently advected eastward. A typical timescale for advection across the South Pacific and into the South Atlantic is 2 years. This eastward procession was previously associated with the concept of an Antarctic Circumpolar Wave (White and Peterson, 1996[40]), whereby pairs of coupled anomalies in SST, sea ice concentration, winds etc propagate eastward around Antarctica. The potential ENSO trigger for such a wave was recognised early (e.g. Peterson and White, 1998[65]), however it is now known that this phenomenon exists only during certain time periods, and is distorted or absent during others (Connolley, 2003[45]).
Despite this, it is important to recognise that the SST anomalies that are advected around the Southern Ocean are subject to further air-sea interaction as they propagate, and will be magnified or diminished as a result. The southeast Pacific is one region very susceptible to ENSO forcing (see above), and Meredith et al. (2007[66]) showed that the phasing of advection of SST anomalies across the South Pacific generally coincides with that of ENSO, such that positive reinforcement of the SST anomalies occurs here. This acts to sustain the anomalies as they propagate eastward. As ENSO-induced anomalies propagate eastward within the ACC, phased changes on comparable timescales occur further south within the subpolar gyres (e.g. Venegas and Drinkwater, 2001[67]).
ENSO is not the only tropical/equatorial phenomenon with a teleconnection to high southern latitudes. The Madden-Julian Oscillation (MJO, Madden and Julian, 1994[68]) is the dominant mode of intraseasonal variability in the tropical atmosphere, and is associated with large-scale convective anomalies that propagate slowly eastward from the Indian Ocean to the western Pacific with a period of approximately 30-70 days. Matthews and Meredith (2004[69]) showed that during the southern winter the MJO has an atmospheric extratropical response that impacts on the surface westerly winds around the 60ºS latitude band, which are the winds that drive the ACC. They were able to show that changes in the ACC were induced in response to tropical variability. The total timescale for MJO to influence ACC transport was of the order of 1-2 weeks.
In addition to teleconnections from lower latitudes influencing the Southern Ocean and Antarctica, it is now known that they can operate in the reverse direction also. Recent studies (e.g. Ivchenko et al., 2004[70]; Blaker et al., 2006[71]) have demonstrated that signals generated in regions such as the Weddell Sea by anomalies in the cover of sea ice or the upper-layers of the ocean can propagate through the Drake Passage to the western Pacific as fast ocean barotropic Rossby waves. The time scale for this propagation is just a few days, and subsequently the signal propagates as an oceanic Kelvin wave along the western boundary and the equator, reaching the equatorial western coast of South America after 2-3 months. This impact on the equatorial regions raises the prospect of a potential high-latitude influence on ENSO, and hence complex coupled ocean-atmosphere feedbacks between the equatorial Pacific and high latitude Southern Ocean. Ongoing research is investigating this possibility.
Studies with a coupled global climate model (Richardson et al., 2005[72]) indicate an inter-hemispheric coupling on the time scale of 10 years. A freshwater anomaly generated in the Southern Ocean inhibits the ventilation of deep waters around Antarctica which causes the deep ocean to warm and the surface to cool. Cooling induces an increase of sea ice thickness and extent which causes cooling of the atmosphere. The cooling signal propagates in the Pacific to the Northern Hemisphere in less than a decade where it affects the North Atlantic Oscillation.
References
- ↑ Rogers, J.C. and Van Loon, H. 1982. Spatial Variability of Sea Level Pressure and 500 mb Height Anomalies over the Southern Hemisphere, Mon. Wea. Rev., 110, 1375-1392.
- ↑ Fyfe, J.C. and Lorenz, D. 2005. Characterizing midlatitude jet variability: Lessons from a simple GCM, Journal of Climate, 18, 3400-3404.
- ↑ Thompson, D.W.J. and Wallace, J.M. 2000. Annular modes in extratropical circulation, Part 1: Month-to-month variability, J. Climate, 13, 1000-1016.
- ↑ Limpasuvan, V. and Hartmann, D.L. 2000. Wave-maintained annular modes of climate variability, Journal of Climate, 13, 4414-4429.
- ↑ Hines K.M., Bromwich, D.H. and Marshall, G.J. 2000. Artificial surface pressure trends in the NCEP–NCAR reanalysis over the Southern Ocean and Antarctica, J. Climate, 13, 3940-3952.
- ↑ Gong, D. and Wang, S. 1999. Definition of Antarctic Oscillation index, Geophys. Res. Lett., 26, 459−462.
- ↑ 7.0 7.1 7.2 Marshall, G. J. 2003. Trends in the Southern Annular Mode from Observations and Reanalyses, Journal of Climate, 16, 4134-4143.
- ↑ Souney, J., Mayewski, P.A., Goodwin, I., Morgan, V. and Van Ommen, T. 2002. A 700-year record of atmospheric circulation developed from the Law Dome ice core, East Antarctica, J. Geophys. Res., 107 (D22), 4608-4616.
- ↑ Jones, J.M. and Widmann, M. 2003. Instrument- and tree-ring-based estimates for the Antarctic Oscillation, J. Clim., 16(21), 3511-3524.
- ↑ Yan, Y., Mayewski, P.A., Kang, S. and Meyerson, E. 2005. An ice core proxy for Antarctic circumpolar wind intensity, Annals of Glaciology, 41, 121-130.
- ↑ IPCC 2007. Climate Change 2007: The Physical Science Basis. Contribution of the Intergovernmental Panel on Climate Change. Cambridge University Press, Cambridge.
- ↑ Thompson, D.W.J., Wallace, J.M. and Hegerl, G.C. 2000. Annular modes in the extratropical circulation: Part II: Trends, J. Climate, 13, 1018-1036.
- ↑ Marshall, G.J. 2002. Analysis of recent circulation and thermal advection in the northern Antarctic Peninsula, Int. J. Climatol., 22,1557-1567.
- ↑ Thompson, D. and Solomon, S. 2002. Interpretation of recent southern hemisphere climate change, Science, 296(5569), 895-899.
- ↑ 15.0 15.1 Kwok, R. and Comiso, J.C. 2002. Southern ocean climate and sea ice anomalies associated with the Southern Oscillation, J. Climate, 15, 487-501.
- ↑ 16.0 16.1 Schneider, D.P., Steig, E.J. and Comiso, J.C. 2004. Recent climate variability in Antarctica from satellite-derived temperature data, J. Climate, 17, 1569-1583.
- ↑ Marshall, G.J. 2007. Half-century seasonal relationships between the Southern Annular Mode and Antarctic temperatures, Int. J. Climatol., 27, 373-383.
- ↑ Genthon, C., Krinner, G. and Sacchettini, M. 2003. Interannual Antarctic tropospheric circulation and precipitation variability, Clim. Dyn., 21, 289-307.
- ↑ Aoki, S. 2002. Coherent sea level response to the Antarctic Oscillation. Geophysical Research Letters, 29(20), 1950, doi:10.1029/2002GL015733.
- ↑ Hughes, C.W., Woodworth, P.L., Meredith, M.P., Stepanov, V., Whitworth, T. and Pyne A.R. 2003. Coherence of Antarctic sea levels, Southern Hemisphere Annular Mode, and flow through Drake Passage, Geophysical Research Letters, 30(9), 1464, doi:10.1029/2003GL017240.
- ↑ Meredith, M.P., Woodworth, P.L., Hughes, C.W. and Stepanov, V. 2004. Changes in the ocean transport through Drake Passage during the 1980s and 1990s, forced by changes in the Southern Annular Mode, Geophysical Research Letters, 31(21), L21305, 10.1029/2004GL021169.
- ↑ Hall, A. and Visbeck, M. 2002. Synchronous variability in the southern hemisphere atmosphere, sea ice, and ocean resulting from the annular mode, Journal of Climate, 15(21), 3043-3057.
- ↑ Lefebvre, W., Goosse, H., Timmermann, R. and Fichefet, T. 2004. Influence of the Southern Annular Mode on the sea ice-ocean system, J. Geophys. Res., 109, C09005, doi:10.1029/2004JC002403.
- ↑ Gille, S.T. 2002. Warming of the Southern Ocean since the 1950s, Science, 295(5558), 1275-1277, doi:10.1126/science.1065863.
- ↑ Liu, J., Curry, J.A. and Martinson, D.G. 2004. Interpretation of recent Antarctic sea ice variability, Geophys. Res. Lett., 31, L02205, doi:10.1029/2003GL018732.
- ↑ Raphael, M.N. 2003. Impact of observed sea-ice concentration on the Southern Hemisphere extratropical atmospheric circulation in summer, J. Geophys. Res., 108, No. D22, 4687 10.1029/2002JD003308.
- ↑ Marshall, G.J. and Connolley, W.M. 2006. The effect of changing Southern Hemisphere winter sea surface temperatures on Southern Annular Mode strength, Geophysical Research Letters, 33 (17), 4, pp. doi 10.1029/2006GL026627.
- ↑ 28.0 28.1 Mo, K.C. and Higgins, R.W. 1998. The Pacific-South America modes and tropical convection during the Southern Hemisphere winter, Mon. Weather Rev., 126, 1581-1596.
- ↑ 29.0 29.1 Yuan, X.J. 2004. ENSO-related impacts on Antarctic sea ice: a synthesis of phenomenon and mechanisms, Antarctic Science, 16(4), 415-425.
- ↑ Karoly, D.J. 1989. Southern Hemisphere circulation features associated with El Niño-Southern Oscillation events, Journal of Climate, 2, 1239-1252.
- ↑ Turner, J. 2004. The El Niño-Southern Oscillation and Antarctica, International Journal of climatology, 24, 1-31.
- ↑ Liu, J.P., Yuan, X.J., Rind, D. and Martinson, D.G. 2002. Mechanism study of the ENSO and southern high latitude climate teleconnections, Geophysical Research Letters, 29, 1679.
- ↑ Lachlan-Cope, T.A. and Connolley, W.M. 2006. Teleconnections between the tropical Pacific and the Amundsen-Bellingshausen Sea: role of the El Niño/Southern Oscillation, Journal of Geophysical Research, 111 [D23], doi:10.1029/2005JD006386.
- ↑ Fogt, R.L. and Bromwich, D.H. 2006. Decadal Variability of the ENSO Teleconnection to the High-Latitude South Pacific Governed by Coupling with the Southern Annular Mode, J. Climate, 19, 979-997.
- ↑ Schneider, D.P. and Steig, E.J. 2008. Ice cores record significant 1940s Antarctic warmth related to tropical climate variability, Proceedings of the National Academy of Sciences of the United States of America, 105(34), 12154-12158.
- ↑ Renwick, J.A. and Revell, M.J. 1999. Blocking over the South Pacific and RossbyWave propagation, Mon.Wea. Rev., 127, 2233-2247.
- ↑ 37.0 37.1 37.2 Yuan, X.J. and Martinson, D.G. 2001. The Antarctic Dipole and its predictability, Geophysical Research Letters, 28(18), 3609-3612.
- ↑ 38.0 38.1 Yuan, X.J. and Martinson, D.G. 2000. Antarctic sea ice extent variability and its global connectivity, Journal of Climate, 13(10): 1697-1717.
- ↑ Gloersen, P. 1995. Modulation of hemispheric ice cover by ENSO events, Nature, 373, 503-505.
- ↑ 40.0 40.1 40.2 40.3 40.4 White, W.B. and Peterson, R. 1996. An Antarctic Circumpolar Wave in surface pressure, wind, temperature, and sea ice extent, Nature, 380, 699-702.
- ↑ Jacobs, G.A. and Mitchell, J.L. 1996. Ocean circulation variations associated with the Antarctic Circumpolar Wave, Geophys.. Res. Let., 23, 2947-2950.
- ↑ Gloersen, P. and White, W.B. 2001. Reestablishing the circumpolar wave in sea ice around Antarctica from one winter to the next, J. Geophys. Res., 106, 4391-4395.
- ↑ White, W.B., Gloersen, P. and Simmonds, I. 2004. Tropospheric response in the Antarctic circumpolar wave along the sea ice edge around Antarctica, J. Clim., 17 (14), 2765-2779.
- ↑ Fischer, H., Traufetter, F., Oerter, H., Weller, R. and Miller, H. 2004. Prevalence of the Antarctic Circumpolar Wave over the last two millenia recorded in Dronning Maud Land ice, Geophys. Res. Lett., 31, L08202, doi:10.1029/2003GL019186.
- ↑ 45.0 45.1 Connolley, W.M. 2003. Long-term variation of the Antarctic Circumpolar Wave, J. Geophys. Res., 108, 8076, doi:10.1029/ 2000JC000380.
- ↑ 46.0 46.1 46.2 Cai, W., Baines, P.G. and Gordon, H.B. 1999. Southern mid- to high-latitude variability, a zonal wavenumber-3 pattern, and the Antarctic circumpolar wave in the CSIRO coupled model, J. Climate, 12, 3087-3104.
- ↑ 47.0 47.1 47.2 Venegas, S.A. 2003. The Antarctic Circumpolar Wave: a combinationof two signals?, Journal of Climate, 16, 2509-2525.
- ↑ White, W.B., Chen, S.C., Allan, R.J. and Stone, R.C. 2002. Positive feedbacks between the Antarctic Circumpolar Wave and the global El Nino-Southern Oscillation Wave, Journal of Geophysical Research Oceans, 107, 3165..
- ↑ Weisse, R., Mikolajewicz, U., Sterl, A. and Drijfhout, S.S. 1999. Stochastically forced variability in the Antarctic Circumpolar Current, J. Geophys. Res., 104(C5), 11,049-11,064.
- ↑ 50.0 50.1 50.2 Turner, J., Leonard, S., Lachlan-Cope, T., and Marshall, G.J. 1998. Understanding Antarctic Peninsula precipitation distribution and variability using a numerical weather prediction model, Ann. Glaciol., 27, 591-596.
- ↑ 51.0 51.1 Jones, D.A. and Simmonds, I. 1993. A climatology of Southern Hemisphere extratropical cyclones, Climate Dynamics, 9, 131-145.
- ↑ 52.0 52.1 52.2 52.3 Hoskins, B.J. and Hodges, K. 2005. A new perspective on southern hemisphere winter storm tracks, Journal of Climate, 18, 4108-4129.
- ↑ 53.0 53.1 53.2 Simmonds, I. and Keay, K. 2000. Variability of Southern Hemisphere extratropical cyclone behaviour, 1958-97, J. Climate, 13(3), 550-561.
- ↑ Sinclair, M.R. 1996. A climatology of anticyclones and blocking for the Southern Hemisphere, Mon. Wea. Rev., 124, 245-263.
- ↑ 55.0 55.1 Simmonds, I.H., Keay, K. and Lim, E. 2003. Synoptic Activity in the Seas around Antarctica, Monthly Weather Review, 131, 272-288.
- ↑ Enomoto, H., and coauthors. 1998. Winter warming over Dome Fuji, east Antarctica and semi-annual oscillation in the atmospheric circulation, J. Geophys. Res., 103, 23103-23111.
- ↑ Hirasawa, N., Nakamura, H. and Yamanouchi, T. 2000. Abrupt changes in meteorological conditions observed at an inland Antarctic station in association with wintertime blocking formation, Geophys. Res. Lett., 27, 1911-1914.
- ↑ Pook, M. and Gibson, T. 1999. Atmospheric blocking and storm tracks during SOP-1 of the FROST Project, Aust. Meteorol. Mag., Special Edition, 51-60.
- ↑ Bertler, N.A.N., Barrett, P.J., Mayewski, P.A., Fogt, R.L., Kreutz, K.J. and Shulmeister, J. 2004. El Niño suppresses Antarctic warming, Geophys. Res. Lett., 31, L15207, doi:10.1029/2004GL020749.
- ↑ Doran, P.T., Priscu, J.C., Lyons, W.B., Walsh, J.E., Fountain, A.G., McKnight, D.M., Moorhead, D.L., Virginia, R.A., Wall, D.H., Clow, G.D., Fritsen, C.H., McKay, C.P. and Parsons, A.N. 2002. Antarctic climate cooling and terrestrial ecosystem response, Nature, 415, 517-520.
- ↑ Cullather, R.I., Bromwich, D.H. and Van Woert, M.L. 1996. Interannual variations in Antarctic precipitation related to El Nino southern oscillation, Journal of Geophysical Research, 101, 19109-19118.
- ↑ Turner, J., Colwell, S.R., Marshall, G.J., Lachlan-Cope, T.A., Carleton, A.M., Jones, P.D., Lagun, V., Reid, P.A. and Iagovkina, S. 2005a. Antarctic climate change during the last 50 years, International Journal of Climatology, 25, 279-294.
- ↑ Meyerson, E.A., Mayewski, P.A., Whitlow, S.I., Meeker, L.D., Kreutz, K.J. and Twickler, M.S. 2002. The extratropical expression of ENSO recorded in a South Pole glaciochemical time series, Annals of Glaciology, 35, 430-436.
- ↑ Li, Z.X., 2000. Influence of Tropical Pacific El Nino on the SST of the Southern Ocean through atmospheric bridge, Geophysical Research Letters, 27 (21), 3505-3508.
- ↑ Peterson, R.G. and White, W.B. 1998. Slow oceanic teleconnections linking the Antarctic Circumpolar Wave with tropical ENSO, Journal of Geophysical Research, 103, 24573-24583.
- ↑ Meredith, M.P., Murphy, E.J. Hawker, E.J., King, J.C. and Wallace, M.I. 2007. On the interannual variability of ocean temperatures around South Georgia, Southern Ocean: forcing by El Niño/Southern Oscillation and the Southern Annular Mode, Deep-Sea Research II, in press.
- ↑ Venegas, S.A. and Drinkwater, M.R. 2001. Sea ice, atmosphere and upper ocean variability in the Weddell Sea, Antarctica, Journal of Geophysical Research, 106(C8), 16,747-16,766.
- ↑ Madden, R.A. and Julian, P.R. 1994. Observations of the 40-50 day tropical oscillation - A review, Monthly Weather Review, 122, 814-837.
- ↑ Matthews, A.J. and Meredith, M.P. 2004. Variability of Antarctic circumpolar transport and the Southern Annular Mode associated with the Madden-Julian Oscillation, Geophysical Research Letters, 31(24), L24312, 10.1029/2004GL021666.
- ↑ Ivchenko, V.O., Zalesny, V.B. and Drinkwater, M.R. 2004. Can the Equatorial Ocean quickly responds to Antarctic sea ice-salinity anomalies?, Geophys. Res. Lett., 31(15), doi: 10.1029/2004GL020472.
- ↑ Blaker, A.T., Sinha, B., Ivchenko, V.O., Wells, N.C. and Zalesny, V.B. 2006. Identifying the roles of the ocean and atmosphere in creating a rapid equatorial response to a Southern Ocean anomaly, Geophys. Res. Lett., 33, L06720, doi:10.1029/2005GL025474.
- ↑ Richardson, G., Wadley, M.R., Heywood, K.J., Stevens, D.P. and Banks, H.T. 2005. Short-term climate response to a freshwater pulse in the Southern Ocean, Geophysical Research Letters, 32, L03702, doi:10.1029/2004GL021586.