Difference between revisions of "Observations of the ice sheet"
(Importing text file) |
(Removed link to Observations of Antarctic Permafrost, which now lives under Observations, data accuracy and tools) |
||
Line 84: | Line 84: | ||
ITASE research reveals high variability in surface mass balance, such that single cores, stakes, and snowpits do not always represent the geographical and environmental characteristics of a local region (Richardson and Holmlund, 1999<ref name="Richardson and Holmlund, 1999">Richardson, C. and Holmlund, P. 1999. Regional and local variability in shallow snow layer depth from a 500 km continuous radar traverse on the polar plateau, central Dronning Maud Land, East Antarctica, ''Annals of Glaciology'', '''29''', 10-16.</ref>; Frezzotti et al., 2004<ref name="Frezzotti et al, 2004">Frezzotti, M., Pourchet, M., Flora, O., Gandolfi, S., Gay, M., Urbini, S., Vincent, C., Becagli, S., Gragnani, R., Proposito, M., Severi, M., Traversi, R., Udisti, R. and Fily, M. 2004. New estimations of precipitation and surface sublimation in East Antarctica from snow accumulation measurements, ''Climate Dynamics'', '''23''', 803-813.</ref>; Spikes et al., 2004<ref name="Spikes et al, 2004">Spikes, V.B., Hamilton, G.S., Arcone, S.A., Kaspari, S. and Mayewski, P.A. 2004. Variability in accumulation rates from GPR profiling on the West Antarctic Plateau, ''Ann. Glaciol.'', '''39''', 238-244.</ref>; Nishio et al., 2002<ref name="Nishio et al, 2002">Nishio, F., Furukawa, T., Hashida, G., Igarashi, M., Kameda, T., Kohno, M., Motoyama, H., Naoki, K., Satow, K., Suzuki, K., Takata, M., Toyama, Y., Yamada, T. and Watanabe, O. 2002. Annual-layer determinations and 167 year records of past climate of H72 ice core in east Dronning Maud Land, Antarcitaca, ''Ann. Glacio.'', '''35''', 471-479.</ref>). For example, Frezzotti et al. (2004<ref name="Frezzotti et al, 2004">Frezzotti, M., Pourchet, M., Flora, O., Gandolfi, S., Gay, M., Urbini, S., Vincent, C., Becagli, S., Gragnani, R., Proposito, M., Severi, M., Traversi, R., Udisti, R. and Fily, M. 2004. New estimations of precipitation and surface sublimation in East Antarctica from snow accumulation measurements, ''Climate Dynamics'', '''23''', 803-813.</ref>) show that spatial surface mass balance variability at sub-kilometre scales (as is typically represented in ice cores) overwhelms temporal variability at the century scale for a low-accumulation site in East Antarctica. Emerging data collected by ITASE and associated deep ice core projects reveals systematic biases in long-term estimates of surface mass balance compared to previous compilations; the biases are presumably related to the small-scale spatial variability (Oerter et al., 1999<ref name="Oerter et al, 1999">Oerter, H., Graf, W., Wilhelms, F., Minikin, A. and Miller, H. 1999. Accumulation studies on Amundsenisen, Dronning Maud Land, Antarctica, by means of tritium, dielectric profiling and stable-isotope measurements: first results from the 1995-96 and 1996-97 field seasons, ''Ann. Glaciol.'', '''29''', 1-9.</ref>; Frezzotti et al., 2004<ref name="Frezzotti et al, 2004">Frezzotti, M., Pourchet, M., Flora, O., Gandolfi, S., Gay, M., Urbini, S., Vincent, C., Becagli, S., Gragnani, R., Proposito, M., Severi, M., Traversi, R., Udisti, R. and Fily, M. 2004. New estimations of precipitation and surface sublimation in East Antarctica from snow accumulation measurements, ''Climate Dynamics'', '''23''', 803-813.</ref>, Magand et al., 2004<ref name="Magand et al, 2004">Magand, O., Frezzotti, M., Pourchet, M., Stenni, B., Genoni, L. and Fily, M. 2004. Climate variability along latitudinal and longitudinal transects in East Antarctica, ''Annals of Glaciology'', '''39''', 351-358.</ref>; Rotschky et al., 2004<ref name="Rotschky et al, 2004">Rotschky, G., Eisen, O., Wilhelms, F., Nixdorf, U. and Oerter, H. 2004. Spatial distribution of surface mass balance on Amundsenisen plateau, Antarctica, derived from ice-penetrating radar studies, ''Annals of Glaciology'', '''39''', 265-270.</ref>). The extensive use, along ITASE traverses, of new techniques like geolocated GPR profiling integrated with core data, provides detailed information on surface mass balance (Richardson and Holmlund, 1999<ref name="Richardson and Holmlund, 1999">Richardson, C. and Holmlund, P. 1999. Regional and local variability in shallow snow layer depth from a 500 km continuous radar traverse on the polar plateau, central Dronning Maud Land, East Antarctica, ''Annals of Glaciology'', '''29''', 10-16.</ref>; Urbini et al., 2001<ref name="Urbini et al, 2001">Urbini, S., Gandolfi, S. and Vittuari, L. 2001. GPR and GPS data integration: examples of application in Antarctica, ''Annali di Geofisca'', '''44''' (4), 687-702.</ref>; Arcone et al., 2005<ref name="Arcone et al, 2005">Arcone, S.A., Spikes, V.B., Hamilton, G. and Mayewski, P.A. 2005. Continuity, vertical resolution and origin of stratigraphy in 400-Mhz short-pulse radar profiles of firn in West Antarctica, ''Annals of Glaciology'', '''39''', 195-200.</ref>; Rotschky et al., 2004<ref name="Rotschky et al, 2004">Rotschky, G., Eisen, O., Wilhelms, F., Nixdorf, U. and Oerter, H. 2004. Spatial distribution of surface mass balance on Amundsenisen plateau, Antarctica, derived from ice-penetrating radar studies, ''Annals of Glaciology'', '''39''', 265-270.</ref>). At some sites stake farm and ice core accumulation rates differ significantly, but isochronal layers in firn, detected with GPR, correlate well with ice core chronologies (Frezzotti et al., 2004<ref name="Frezzotti et al, 2004">Frezzotti, M., Pourchet, M., Flora, O., Gandolfi, S., Gay, M., Urbini, S., Vincent, C., Becagli, S., Gragnani, R., Proposito, M., Severi, M., Traversi, R., Udisti, R. and Fily, M. 2004. New estimations of precipitation and surface sublimation in East Antarctica from snow accumulation measurements, ''Climate Dynamics'', '''23''', 803-813.</ref>). Several GPR layers within the upper 100 m of the surface have been surveyed over continuous traverses of 5,000 km and can be used as historical benchmarks to study past accumulation rates (Spikes et al., 2004<ref name="Spikes et al, 2004">Spikes, V.B., Hamilton, G.S., Arcone, S.A., Kaspari, S. and Mayewski, P.A. 2004. Variability in accumulation rates from GPR profiling on the West Antarctic Plateau, ''Ann. Glaciol.'', '''39''', 238-244.</ref>). | ITASE research reveals high variability in surface mass balance, such that single cores, stakes, and snowpits do not always represent the geographical and environmental characteristics of a local region (Richardson and Holmlund, 1999<ref name="Richardson and Holmlund, 1999">Richardson, C. and Holmlund, P. 1999. Regional and local variability in shallow snow layer depth from a 500 km continuous radar traverse on the polar plateau, central Dronning Maud Land, East Antarctica, ''Annals of Glaciology'', '''29''', 10-16.</ref>; Frezzotti et al., 2004<ref name="Frezzotti et al, 2004">Frezzotti, M., Pourchet, M., Flora, O., Gandolfi, S., Gay, M., Urbini, S., Vincent, C., Becagli, S., Gragnani, R., Proposito, M., Severi, M., Traversi, R., Udisti, R. and Fily, M. 2004. New estimations of precipitation and surface sublimation in East Antarctica from snow accumulation measurements, ''Climate Dynamics'', '''23''', 803-813.</ref>; Spikes et al., 2004<ref name="Spikes et al, 2004">Spikes, V.B., Hamilton, G.S., Arcone, S.A., Kaspari, S. and Mayewski, P.A. 2004. Variability in accumulation rates from GPR profiling on the West Antarctic Plateau, ''Ann. Glaciol.'', '''39''', 238-244.</ref>; Nishio et al., 2002<ref name="Nishio et al, 2002">Nishio, F., Furukawa, T., Hashida, G., Igarashi, M., Kameda, T., Kohno, M., Motoyama, H., Naoki, K., Satow, K., Suzuki, K., Takata, M., Toyama, Y., Yamada, T. and Watanabe, O. 2002. Annual-layer determinations and 167 year records of past climate of H72 ice core in east Dronning Maud Land, Antarcitaca, ''Ann. Glacio.'', '''35''', 471-479.</ref>). For example, Frezzotti et al. (2004<ref name="Frezzotti et al, 2004">Frezzotti, M., Pourchet, M., Flora, O., Gandolfi, S., Gay, M., Urbini, S., Vincent, C., Becagli, S., Gragnani, R., Proposito, M., Severi, M., Traversi, R., Udisti, R. and Fily, M. 2004. New estimations of precipitation and surface sublimation in East Antarctica from snow accumulation measurements, ''Climate Dynamics'', '''23''', 803-813.</ref>) show that spatial surface mass balance variability at sub-kilometre scales (as is typically represented in ice cores) overwhelms temporal variability at the century scale for a low-accumulation site in East Antarctica. Emerging data collected by ITASE and associated deep ice core projects reveals systematic biases in long-term estimates of surface mass balance compared to previous compilations; the biases are presumably related to the small-scale spatial variability (Oerter et al., 1999<ref name="Oerter et al, 1999">Oerter, H., Graf, W., Wilhelms, F., Minikin, A. and Miller, H. 1999. Accumulation studies on Amundsenisen, Dronning Maud Land, Antarctica, by means of tritium, dielectric profiling and stable-isotope measurements: first results from the 1995-96 and 1996-97 field seasons, ''Ann. Glaciol.'', '''29''', 1-9.</ref>; Frezzotti et al., 2004<ref name="Frezzotti et al, 2004">Frezzotti, M., Pourchet, M., Flora, O., Gandolfi, S., Gay, M., Urbini, S., Vincent, C., Becagli, S., Gragnani, R., Proposito, M., Severi, M., Traversi, R., Udisti, R. and Fily, M. 2004. New estimations of precipitation and surface sublimation in East Antarctica from snow accumulation measurements, ''Climate Dynamics'', '''23''', 803-813.</ref>, Magand et al., 2004<ref name="Magand et al, 2004">Magand, O., Frezzotti, M., Pourchet, M., Stenni, B., Genoni, L. and Fily, M. 2004. Climate variability along latitudinal and longitudinal transects in East Antarctica, ''Annals of Glaciology'', '''39''', 351-358.</ref>; Rotschky et al., 2004<ref name="Rotschky et al, 2004">Rotschky, G., Eisen, O., Wilhelms, F., Nixdorf, U. and Oerter, H. 2004. Spatial distribution of surface mass balance on Amundsenisen plateau, Antarctica, derived from ice-penetrating radar studies, ''Annals of Glaciology'', '''39''', 265-270.</ref>). The extensive use, along ITASE traverses, of new techniques like geolocated GPR profiling integrated with core data, provides detailed information on surface mass balance (Richardson and Holmlund, 1999<ref name="Richardson and Holmlund, 1999">Richardson, C. and Holmlund, P. 1999. Regional and local variability in shallow snow layer depth from a 500 km continuous radar traverse on the polar plateau, central Dronning Maud Land, East Antarctica, ''Annals of Glaciology'', '''29''', 10-16.</ref>; Urbini et al., 2001<ref name="Urbini et al, 2001">Urbini, S., Gandolfi, S. and Vittuari, L. 2001. GPR and GPS data integration: examples of application in Antarctica, ''Annali di Geofisca'', '''44''' (4), 687-702.</ref>; Arcone et al., 2005<ref name="Arcone et al, 2005">Arcone, S.A., Spikes, V.B., Hamilton, G. and Mayewski, P.A. 2005. Continuity, vertical resolution and origin of stratigraphy in 400-Mhz short-pulse radar profiles of firn in West Antarctica, ''Annals of Glaciology'', '''39''', 195-200.</ref>; Rotschky et al., 2004<ref name="Rotschky et al, 2004">Rotschky, G., Eisen, O., Wilhelms, F., Nixdorf, U. and Oerter, H. 2004. Spatial distribution of surface mass balance on Amundsenisen plateau, Antarctica, derived from ice-penetrating radar studies, ''Annals of Glaciology'', '''39''', 265-270.</ref>). At some sites stake farm and ice core accumulation rates differ significantly, but isochronal layers in firn, detected with GPR, correlate well with ice core chronologies (Frezzotti et al., 2004<ref name="Frezzotti et al, 2004">Frezzotti, M., Pourchet, M., Flora, O., Gandolfi, S., Gay, M., Urbini, S., Vincent, C., Becagli, S., Gragnani, R., Proposito, M., Severi, M., Traversi, R., Udisti, R. and Fily, M. 2004. New estimations of precipitation and surface sublimation in East Antarctica from snow accumulation measurements, ''Climate Dynamics'', '''23''', 803-813.</ref>). Several GPR layers within the upper 100 m of the surface have been surveyed over continuous traverses of 5,000 km and can be used as historical benchmarks to study past accumulation rates (Spikes et al., 2004<ref name="Spikes et al, 2004">Spikes, V.B., Hamilton, G.S., Arcone, S.A., Kaspari, S. and Mayewski, P.A. 2004. Variability in accumulation rates from GPR profiling on the West Antarctic Plateau, ''Ann. Glaciol.'', '''39''', 238-244.</ref>). | ||
− | |||
− | |||
==References== | ==References== | ||
<references /> | <references /> | ||
[[Category:Observations, data accuracy and tools]] | [[Category:Observations, data accuracy and tools]] | ||
[[Category:The Antarctic ice sheet]] | [[Category:The Antarctic ice sheet]] |
Revision as of 14:55, 6 August 2014
- This page is part of the topic Observations, data accuracy and tools
Contents
Introduction
Since the earliest exploration of Antarctica scientists have visited the ice plateau in search of new discoveries. Despite the logistic and physical difficulties imposed by the environment and weather, field-based observations have been made throughout Antarctica, and have proved invaluable in explaining the dynamic character of this body of ice. An era of international fieldwork, sparked by the IGY of 1957-58, has continued to the recent International Polar Year of 2007-08. In the intervening decades, glaciological fieldwork adopted new technologies and revealed behaviour that could not have been observed by any other means. Some measurements can now be made over wide areas from satellites or aircraft, but direct in-situ observations are still needed to provide calibration and validation for these surveys. Moreover, fieldwork provides detailed information about the processes that operate to change the Antarctic environment. The combination of fieldwork, aircraft, and satellite measurements has revealed changes in Antarctica on many scales and given a better understanding of their causes.
Surface elevation
Oversnow traverses provided the first indications of the surface shape of the ice sheet. The existence of broad, flat ice shelves bounded by tall mountain ranges that retained a high, frigid and harsh polar plateau played into the strategies used by explorers to reach the South Pole. However, such sparse sampling could never hope to attain sufficient coverage of the ice sheet to detect its shape in detail. In the mid-1970s, random sampling of the surface from radar altimeters onboard balloons released to circulate above the continent at constant atmospheric pressure greatly increased the mapping of the ice sheet’s surface elevation (Levanon et al., 1977[1]). Radar altimeters use the ranging capability of radar to measure the surface topographic profile beneath the sensor by measuring the time interval between the transmission and reception of very short electromagnetic pulses. These data showed the ice sheet surface topography contained a number of separate domes in East Antarctica and that this ice sheet was much higher than the West Antarctic ice sheet (Figure 1.2). With this information, the drainage basins of individual outlet glaciers could begin to be defined (Figure 1.3).
Radar altimeters onboard satellites established systematically repeated surface elevation observations of the earth. To date, most spaceborne radar altimeters have been wide-beam (pulse-limited) systems operating from low Earth orbits. Radar altimetry data of Antarctica has been collected since 1978 from a variety of instruments including Seasat (1978), Geosat (1985-1990), ERS-1 (1991-1996) and ERS-2 (since 1995), and Envisat (since 2002). Seasat reached only to 72º S whereas subsequent missions have increased coverage to 81.5º S. The measurement accuracy of such systems is sub-metre across the smoother ice sheet interior, but degrades to a few metres as the surface slope increases, especially nearer the coast and in mountainous regions. The future availability of satellite altimetry observations is assured with new missions such as ESA’s Sentinel satellite series. ESA’s CryoSat mission will also provide new data from the SIRAL instrument specifically designed for ice sheet and sea ice observations.
In addition to radar altimetry, the first satellite laser altimeter was launched in 2003 onboard the ICESat satellite. The Geoscience Laser Altimeter System (GLAS) is the sole instrument on the Ice, Cloud, and land Elevation Satellite (ICESat) (Figure 2.16). A laser altimeter has the advantage of ranging directly to the surface without penetrating the upper snow layers, but requires cloud-free conditions. Microwave radar is unaffected by clouds, but penetrates several metres into the snow (Legresy and Remy, 1997[2]; Arthern et al., 2001[3]). Alteration of the snowpack by weather and climate could change the depth of penetration, with a risk that this is mistaken for a real change in the surface elevation. Recent studies have corrected for this effect using an empirical, location-dependent correlation between penetration depth and backscattered power (Wingham et al., 1998[4]; Zwally et al., 2005[5]), although some residual error may be unavoidable (Alley et al., 2007[6]). Both radar and laser altimetry can be affected by changes in the density of the upper layers of the snowpack, so these must be estimated separately or corrected for (Arthern and Wingham, 1998[7]; Zwally et al., 2005[5]).
The primary objective of the ICESat mission is to measure ice sheet elevations and changes in elevation through time, with a precision of a few centimetres, surpassing that achieved with radar altimetry. Secondary objectives include measurement of cloud and aerosol height profiles, land elevation and vegetation cover, and sea ice thickness (Schutz et al., 2005[8]). The southern limit of ICESat data is 86º S. The increase in surface topographic detail in these data is illustrated in Figure 1.3 by noting the smoother apparent surface in the region closest to the South Pole; a visual artifact caused by the absence of precise altimetric data in this region.
Most modern airborne geophysical measurement systems operated in Antarctica include a laser altimeter instrument. These have provided independent validation of ICESat data. Their primary function is to focus on regional studies and refine the spatial pattern of elevation change, as indication of ice-sheet or outlet glacier thickness changes.
Satellite altimeter systems have the drawback that their data usually do not extend away from the sub-satellite ground track. ICESat’s GLAS system can be pointed off-nadir, but these occasions are rare. Photoclinometry, or shape from shading interpolated between precisely measured elevation profiles by using optical imagery (Landsat, ASTER and MODIS). These systems collect images of the surface using reflected sunlight in a variety of spectral bands. Because most of Antarctica is snow covered and the albedo of snow at any given wavelength is relatively constant, the brightness variations of ice-sheet imagery are predominantly related to the surface slope in the direction of the solar illumination (Bindschadler and Vornberger, 1998[9]). This relationship allows the image to be used to interpolate surface elevations between satellite altimetry profiles and provide more complete and accurate elevation fields.
A more traditional use of optical imagery to produce elevations is stereo photogrammetry. This can be problematic over ice sheets where distinct features visible in modest-resolution imagery can be uncommon, but with the recent addition of higher spatial-resolution sensors such as ASTER and SPOT-5, the technique is now more often successful. A dedicated set of SPOT stereo images has been acquired around the Antarctica perimeter and elevation data are being produced as users request them.
Bed elevation and ice thickness
As little as fifty years ago, relatively few traverses had been made across the interior of Antarctica, and the thickness of the ice was uncertain over much of the continent. Ice thickness can be determined by the seismic method of detonating explosives and recording the delay before arrival of echoes from the base. This can reveal details of the rock or sediment beneath the ice, as well as the ice thickness (Blankenship et al., 1986[10]). A similar technique using radar, rather than sound waves can be performed from the ground (e.g. Conway et al., 1999[11]; Catania et al., 2006[12]), or from aircraft (Robin et al., 1970[13]; Mae and Yoshida, 1987[14]; Siegert et al., 2005a[15]).
Airborne radar (see section 2.1.2.8), sometimes flown by airplanes based at remote field camps, is now the principle method of determining ice thickness (Figure 2.21). Present technology allows the surface of the underlying rock and sediment to be measured to a vertical precision of tens of metres. Interpolating the rugged bed topography between surveyed tracks remains difficult, and even now there are large regions of Antarctica where few measurements have been recorded. Nevertheless, compiling data from many survey flights can give a good estimate of the volume of ice stored in Antarctica. The most recent compilation of these data is BEDMAP (Lythe et al., 2000[16]) and BEDMAP-2 is underway. Figure 1.5 is a visual illustration of the Antarctic bed elevation field that has resulted from this data compilation. From these data, the total volume of the Antarctic ice sheet is calculated as equivalent to 57 m of sea level. Of this, 52 m is locked away in the thick ice of East Antarctica, and around 5 m is stored in the more dynamic ice sheet covering West Antarctica (Lythe et al., 2000[16]).
Several areas of Antarctica have recently benefited from focused airborne-geophysical surveys. Coastal Dronning Maud Land (Steinhage et al., 1999[17]), Thwaites Glacier (Holt et al., 2006[18]), Pine Island Glacier (Vaughan et al., 2006[19]) have been surveyed in detail. These and similar surveys reveal the amounts of ice present. They also characterise deep troughs in the underlying bed that make some regions more vulnerable than others to rapid change. Furthermore they provide geometric boundary conditions needed for modeling the evolution of the ice sheet.
Velocity
In-situ observations of ice-flow can be made by repeatedly surveying the position of marker poles carried along by the ice (e.g. Naruse, 1979[20]). Nowadays this same direct approach makes use of precise locations derived from GPS. Modern dual-frequency GPS receivers and post-processing of data can give positional accuracy of centimetres or better (King, 2004[21]). The movement of AWS over time along the ice sheets, which have GPS on-board, can also provide invaluable information about ice sheet velocity. Ferrell AWS, located on the Ross Ice Shelf, is estimated to have moved approximately 0.65 - 0.75 km/year over a period from 1979 to 2003. Ice motion is almost everywhere greater than a metre per year, so surface velocities are measured to within one percent in a single year. For faster flowing ice, the relative accuracy is even higher. Point velocities measured in this way provide constraints to calibrate remote sensing methods such as feature tracking or satellite radar interferometry (discussed below).
Features seen from space can serve the same role as surveyed marker poles or GPS antennas. Sequential imagery, once coregistered, offer the opportunity to measure the surface motion of features identified on multiple images. Image coregistration is simplified when there is exposed rock visible. When not, long-wavelength surface forms can be used, because these are surface expressions of fixed basal undulations and, therefore, also fixed in space. Image processing techniques are then employed to first filter out the higher wavelength features and then cross-correlate static long-wavelength features in multiple images. Once coregistered, the same cross-correlation techniques are employed to determine surface feature motion (Scambos et al., 1992[22]). Most often applied to sequential Landsat imagery with a 30 m pixel resolution, the errors in measured displacements are 2-3 pixels with roughly equal parts random error associated with tracking individual features, and systematic error associated with image coregistration.
The optical imagery record began in earnest in 1972 with the launch of ERTS-1, which became the first of the Landsat series. Earlier and recently declassified satellite photographs have extended a sparse data set back to 1960, soon after the first year that satellites were used for military and national security purposes. Spatial resolution, temporal coverage and radiometric sensitivity have steadily improved to the present, when sub-metre pixel resolution, near daily coverage and extraordinary surface detail can be obtained. However, not all of these are available from any single sensor, and the use of the data will help determine what sensor is most suitable.
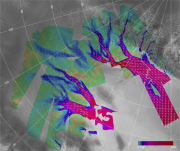
Much information about land ice and snow is obtained from SAR imagery, in particular from SAR Interferometry (InSAR) techniques. This method, which uses phase differences in returned radar signals, is able to measure centimetric changes in surface displacement over very wide areas (Figure 2.23). These new techniques have provided information to monitor glacier discharge (Pritchard and Vaughan, 2007[23]), map grounding lines (Gray et al., 2002[24]; Yamanokuchi et al., 2005[25]), contribute to mass balance studies (Rignot and Thomas, 2002[26]), determine glacier and ice sheet motion (Fischer et al., 2003[27]) and monitor propagation of ice shelf rifts (Fricker et al., 2002[28]). In contrast, an approach taken in matching the amplitudes of SAR images can be used in correlating scenes over a long time period. Nakamura et al. (2007[29]) applied the techniques to the Shirase glacier, one of the fastest-moving ice streams in the Antarctica, and obtained the seasonal variation of velocity near the grounding line, that is the highest in summer and lowest in winter.
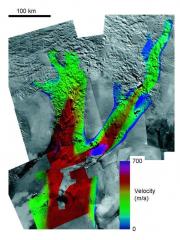
Repeated measurements of horizontal velocity using the GPS technique have shown that the speed of ice streams can change on very short timescales. The speed of ice tens or hundreds of kilometres inland can vary from hour to hour, responding to forcing by the tides (Bindschadler et al., 2003[30]). Other areas respond differently to tides. Flow speed on the Rutford Ice Stream follows a 14 day cycle (Gudmundsson, 2006[31]). Analysing the response of ice streams to known tidal forcing has opened up a new way of studying the friction that impedes the motion of ice.
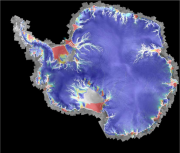
A meaningful diagnostic of the ice sheet state is to calculate the “balance velocity” and compare it with the measured surface velocity. The balance velocity is calculated from the ice thickness, accumulation pattern and flow direction (defined as the direction of maximum surface gradient). It is neither a measured, nor an actual velocity, but corresponds to the depth-averaged velocity at any point that is required to transport all the mass accumulated upstream of that point. If the actual velocity equals the balance velocity everywhere, then the shape of the ice sheet remains unchanged. Thus, balance velocity becomes a convenient way of comparing the geometric shape and meteorological input to the ice speed. Figure 1.6 presents the continental pattern of balance velocity. It compares favourably to the surface speed (Fig 2.24) indicating that a majority of the ice sheet is near equilibrium. Areas of change, including areas changing very rapidly, do occur and will be discussed in Chapter 4.
Mass balance
The overall condition of an ice sheet is usually described as its “mass balance”. It is a fundamental expression of the ice sheet’s health. Mass balance is the sum of all mass inputs to the ice sheet, such as accumulation, minus all mass removals, such as melting. It expresses the rate of growth or shrinkage of the ice sheet and relates directly to the amount of mass transferred to the oceans to affect sea level. Ice leaving the grounded region of the ice sheet affects sea level immediately, even if it remains attached to the ice sheet in the form of a floating ice shelf. Icebergs calved from floating ice shelves and sub-ice shelf melting remove mass from the ice sheet, but do not alter sea level. Sometimes ice shelf mass changes are included in mass balance calculations, because they reflect changes in ice sheet volume, but care must be taken not to equate these mass balance quantities to Antarctica’s contribution to sea level without removing the ice shelf component.
There are currently three independent methods of measuring changes in the mass of the grounded portion of the ice sheet: satellite altimetry, ice-budget, and gravity surveys. All are subject to errors from different sources: principally, snow compaction and electromagnetic penetration for altimetry; thickness errors and accumulation errors for the ice-budget; unmodelled postglacial rebound and atmospheric effects for gravity surveys. The problem is over-determined (three methods for measuring one number) and each of the methods has errors that are (more or less) independent of the others. This means that a better assessment of the state of balance of the ice sheet should be possible by combining all the observations. There are problems doing this, because all the measurements are recorded at different locations, and over different time intervals. A promising approach is to use data assimilation methods similar to those used by numerical weather prediction centres to produce daily weather forecasts (Arthern and Hindmarsh, 2006[33]). This should allow a better analysis of the present-day changes, and opens up the possibility of forecasting the evolution of the ice sheet over the coming decades. Even before such formal methods are applied, comparison of the three approaches provides a valuable consistency check.
The concept of mass balance can also be applied to any portion of the ice sheet. To measure the amount of ice transported, the thickness and the profile of velocity through the column are needed. If the base is slippery, or the ice is afloat, an assumption that ice speed is constant at all depths throughout the column is appropriate. Then the ice flux is simply the product of surface velocity and thickness. For ice thickness of the order of kilometres, the depth error from radar surveys is of the order of one percent, and dominates over the velocity error. If shearing is present in the column it must be corrected for, and this can introduce errors larger than one percent in the flux. The accuracy that can be achieved for the flux leaving any particular drainage basin is typically of the order of ten percent of the turnover (e.g. Fricker et al., 2000[34]; Rignot and Thomas, 2002[26]).
Since measurements began, the overall mass balance of the ice sheet (i.e., the rate of change of the total mass of ice held within it) has been slightly negative, but with large uncertainties. This belies the enormous regional variability of mass changes that express different dynamic characteristics and responses to different climate forcings. Some signals, such as thinning of the Amundsen Sea sector in West Antarctica, are common to all three approaches, and this agreement provides a strong confirmation that this part of Antarctica is contributing to sea level rise. Meanwhile East Antarctica seems close to balance, or thickening slightly, according to these independent assessments.
Point measurements of elevation change, achieved by repeat GPS positioning, can also contribute to mass balance studies (Smith et al., 1998[35]; Hamilton, 2005[36]; Wendt et al., 2009[37]). The vertical component of position derived by GPS is less accurate than the horizontal. The vertical motion of the marker poles must be corrected for along-slope advection, gradients of snow accumulation, and snow compaction. Placing the markers at the bottom of boreholes drilled through the upper layers of the ice sheet, where most of the density changes occur, can lessen the impact of variable snow compaction on these measurements (Hamilton, 2005[36]). The submergence velocity of markers, compared with the long-term rate of snow accumulation (from ice cores), provides a local estimate of the state of balance of the ice sheet.
Ice shelves
Ice shelves, the floating fringe of the ice sheet, are often overlooked because of the absence of a direct impact on sea level, however they have a very important indirect effect. Ice shelves impart forces on grounded glaciers and ice streams, slowing the delivery of ice to the ocean (Thomas, 1973[38]). This means that collapse or melting of a floating ice shelf can trigger a subsequent rise in sea level, as glaciers accelerate when this force is removed. This force is determined not only by the size, lateral extent and temperature of the ice shelf, but also its shape (Walker and Holland, 2007[39]). Drilling allows temperature profiles through the ice to be recorded, and samples of the ice to be recovered for mechanical tests of strength and deformation strength (e.g. Rist et al., 2002[40]). Local mass balance assessments are important, as melt rates at the base of ice shelves can reach several metres a year. Drilling provides access to the oceanographic environment beneath the ice shelf, so that the processes that control melting from the base can be investigated (Nicholls and Jenkins, 1993[41]; Craven et al., 2004[42]). Phase-sensitive radar can be used to measure thinning rates (Corr et al., 2002[43]). This can provide a direct estimate of the basal melt-rate with an accuracy of a few centimetres per year, revealing whether the ice shelf is in a steady state (Jenkins et al., 2006[44]). GPS observations can record the flow velocities of the floating ice, and how it is changing (King et al., 2007[45]). These in-situ observations provide an important test of melt rates, flow rates and thinning rates observed using satellite.
The very definition of where the ice shelves begin needs quantification, and its sensitivity to local ice thickness changes lends it to be an important diagnostic observable. The transition from grounded ice to floating occurs at the “grounding line”. It can be mapped with optical imagery by noting the change in surface roughness: floating ice is smoother due to the absence of basal friction, which supports smaller spatial scale surface undulations on grounded ice. Vertical flexing of the grounding line also can be observed either by interferometric SAR analysis or repeat laser altimetry (Vaughan, 1995[46]; Padman and Fricker, 2005[47]).
The sensitivity of the grounding line to thickness changes results from a shallow basal slopes. Relatively small changes of a few metres in thickness are effectively amplified by many orders of magnitude, depending on the bed slope, to horizontal displacements of hundreds of metres, or even kilometres. In many locations, the grounding line is extremely complex, and patches of ephemerally grounded ice must be included in the observations.
Subglacial hydrology
Ice flow rates in the faster moving ice streams and glaciers of Antarctica are orders of magnitude greater than can be explained by ice deformed by gravitational forces. The additional speed is the result of basal sliding and directly related to lubrication at the ice-bed interface. The degree of lubrication is controlled by the presence of water and sediment (glacial till) underneath the ice. A supply of water from melting at the base of the ice sheet can pressurise subglacial water to the point that the ice is close to flotation. This lessens the load on the underlying sediment and allows it to deform easily, lubricating the sliding. Drag from the edge can be comparable to drag from beneath, even when the width of an ice stream greatly exceeds its thickness. The energy that is needed for melting is provided partly by geothermal heat, and partly by frictional heating (Raymond et al., 2001[48]). Frictional heating is greater for faster sliding, but is also modulated by the degree of lubrication, so a feedback loop links melting to lubrication, speed, friction, and further melting. Depending upon the environment, this loop may reinforce itself, so that ice streams accelerate or decelerate rapidly. In other circumstances variations in velocity are muted, especially if other physical controls, such as trough geometry, limit the margin migration (Raymond et al., 2001[48]). Field measurements have shown that ice streams can flow steadily, have episodes of rapid flow, or shut down completely, depending on details of supply, storage and transport of water and sediment (Retzlaff and Bentley, 1993[49]; Stokes et al., 2007[50]).
In addition to the direct observations of surface velocity (discussed earlier), seismic methods have illuminated some of the processes that control ice stream flow. Although the base of ice streams tend to be well lubricated, the importance of small areas of concentrated friction has been identified by seismicity characteristic of their stick-slip motion (Anandakrishnan and Alley, 1994[51]). These ‘sticky spots’ provide significant retardation to the flow of ice and have a number of possible causes, including reduction of basal water pressure by freezing, channel formation, or redirection of subglacial water flow (recently reviewed by Stokes et al., 2007[50]). Analysis of waveforms reflected from the bed during active seismic sounding can reveal information about whether the sediments are mobile and fluidized, or whether they are lodged and strong enough to support large shear stress retarding the flow of ice (Smith, 2007[52]).
Understandably, there are not many direct observations from beneath the ice streams, but in several places access to the bed has been achieved by hot-water drilling. Video cameras lowered down the borehole (Carsey et al., 2002[53]) have revealed clear ice at the bottom of Kamb ice stream, supporting the interpretation that basal freezing may have contributed to its shutdown around 140 years ago (Vogel et al., 2005[54]). Underneath the ice stream, the camera revealed a water layer 1.6 metres in depth at one site, but just centimetres or less at another. The water flux and linkages between such water cavities are important in determining the basal water pressure and this, in turn, affects the amount of lubrication (Christoffersen and Tulaczyk, 2003[55]; Vogel et al., 2005[54]). The route taken by subglacial water flow is sensitive to surface and bed topography, and changes in surface slope that alter this routing may affect the water pressures, and hence the flow of ice (Alley et al., 1994[56]).
The discovery of thicker reservoirs of subglacial water has led to more direct inference from repeat satellite observations that large volumes of water move between subglacial lakes (Gray et al., 2005[57]; Wingham et al., 2006b[58]; Fricker et al., 2007[59]) and that these larger volumes of water transfer change ice flow rates. These build on a series of discoveries that flow rates change on a wealth of time scales from minutes to millennia. A directed programme of fieldwork has illuminated many causes of this fast and changeable flow (e.g. Alley and Bindschadler, 2001[60]; Bindschadler, 2006[61]). Much of this dynamism can ultimately be traced to the slipperiness of basal sediment pressurised by meltwater.
Mass balance data from ice cores
Ice core records offer a valuable tool to assess natural variability and recent trends in snow accumulation. Climate model predictions lead to a general expectation of an increase in Antarctic precipitation with projected warming. Such an increase acts to offset sea level increases arising from mass loss. Recent results, based on records from ice cores, snow pits and stakes suggest an absence of a warming signal in precipitation over the last 50 years (Monaghan et al., 2006a[62]), with no significant changes in overall precipitation. The data do show, however, a large temporal and spatial variability that underscores the need for mass balance studies to include long-term records from a number of sites.
ITASE research reveals high variability in surface mass balance, such that single cores, stakes, and snowpits do not always represent the geographical and environmental characteristics of a local region (Richardson and Holmlund, 1999[63]; Frezzotti et al., 2004[64]; Spikes et al., 2004[65]; Nishio et al., 2002[66]). For example, Frezzotti et al. (2004[64]) show that spatial surface mass balance variability at sub-kilometre scales (as is typically represented in ice cores) overwhelms temporal variability at the century scale for a low-accumulation site in East Antarctica. Emerging data collected by ITASE and associated deep ice core projects reveals systematic biases in long-term estimates of surface mass balance compared to previous compilations; the biases are presumably related to the small-scale spatial variability (Oerter et al., 1999[67]; Frezzotti et al., 2004[64], Magand et al., 2004[68]; Rotschky et al., 2004[69]). The extensive use, along ITASE traverses, of new techniques like geolocated GPR profiling integrated with core data, provides detailed information on surface mass balance (Richardson and Holmlund, 1999[63]; Urbini et al., 2001[70]; Arcone et al., 2005[71]; Rotschky et al., 2004[69]). At some sites stake farm and ice core accumulation rates differ significantly, but isochronal layers in firn, detected with GPR, correlate well with ice core chronologies (Frezzotti et al., 2004[64]). Several GPR layers within the upper 100 m of the surface have been surveyed over continuous traverses of 5,000 km and can be used as historical benchmarks to study past accumulation rates (Spikes et al., 2004[65]).
References
- ↑ Levanon, N., Julian, P.R. and Suomi, V.E. 1977. Antarctic topography from balloons, Nature, 268, 514-516, doi:10.1038/268514a0.
- ↑ Legresy, B. and Remy, F. 1997. Altimetric observations of surface characteristics of the Antarctic ice sheet, J. Glaciol., 43(144), 265-275.
- ↑ Arthern, R.J., Wingham, D.J. and Ridout, A.L. 2001. Controls on ERS altimeter measurements over ice sheets: Footprint-scale topography, backscatter fluctuations, and the dependence of microwave penetration depth on satellite orientation, J. Geophys. Res., 106(D24), 33,471-33,484.
- ↑ Wingham, D.J., Ridout, A.L., Scharroo, R., Arthern, R.J. and Schum, C.K. 1998, Antarctic elevation change from 1990 to 1996, Science, 282, 456-458, (doi:10.1126/science.282.5388.456).
- ↑ 5.0 5.1 Zwally, H.J., Giovinetto, M., Li, J., Cornejo, H., Beckley, M., Brenner, A., Saba, J. and Yi, D. 2005. Mass changes of the Greenland and Antarctic ice sheets and shelves and contributions to sea-level rise: 1992-2002, Journal of Glaciology, 51(175), 509-527.
- ↑ Alley, R.B., Anandakrishnan, S., Dupont, T.K., Parizek, B.R. and Pollard, D., 2007, Effect of sedimentation on ice-sheet grounding-line stability. Science, 315, 1838-1841.
- ↑ Arthern, R.J. and Wingham, D.J. 1998. The Natural Fluctuations of Firn Densification and Their Effect on the Geodetic Determination of Ice Sheet Mass Balance, Climatic Change, 40, 605-624.
- ↑ Schutz, B.E., Zwally, H.J., Shuman, C.A., Hancock, D. and Dimarzio, J.P. 2005. Overview of the ICESat Mission, Geophys. Res. Lett., 32, L21S01, doi:10.1029/2005GL024009.
- ↑ Bindschadler, R.A. and Vornberger, P.L. 1998. Changes in West Antarctic ice sheet since 1963 from Declassified Satellite Photography, Science, 279, 689-692.
- ↑ Blankenship, D.D., Bentley, C.R., Rooney, S.T. and Alley, R.B. 1986. Seismic measurements reveal a saturated porous layer beneath an active Antarctic ice stream, Nature, 322, 54-57.
- ↑ Conway, H., Hall, B.L., Denton, G.H., Gades, A.M., Waddington, E.D. 1999. Past and future grounding-line retreat of the West Antarctic Ice Sheet. Science 286:280-283.
- ↑ Catania, G.A., Conway, H., Raymond, C.F. and Scambos, T.A. 2006. Evidence for floatation or near floatation in the mouth of Kamb Ice Stream, West Antarctica, prior to stagnation, J. Geophys. Res., 111, F01005, doi:10.1029/2005JF000355.
- ↑ Robin, G. De Q., Swithinbank, C.W.M. and Smith, B.M.E. 1970. Radio echo exploration of the Antarctic ice sheet, Int. Assoc. Sci. Hydrol. Publ., 86, 97-115.
- ↑ Mae, S. and Yoshida, M. 1987. Airborne radio echo-sounding in Shirase Glacier drainage basin, Antarctica, Ann. Glaciol., 9, 160-165.
- ↑ Siegert, M.J., Pokar, M., Dowdeswell, J.A. and Benham, T. 2005a. Radio-echo layering in West Antarctica: a spreadsheet dataset, Earth Surf. Process. Landforms, 30, 1583-1591.
- ↑ 16.0 16.1 Lythe, M.B., Vaughan, D.G. and the BEDMAP Consortium. 2000. BEDMAP - bed topography of the Antarctic. 1:10,000,000 scale map. BAS (Misc) 9. Cambridge, British Antarctic Survey.
- ↑ Steinhage, D., Nixdorf, U., Meyer, U. and Miller, H. 1999. New maps of the ice thickness and subglacial topography in Dronning Maud Land, Antarctica, determined by means of airborne radio echo sounding, Ann. Glaciol., 29, 267-272.
- ↑ Holt, J.W., Blankenship, D.D., Morse, D.L., Young, D.A., Peters, M.E., Kempf, S.D., Richter, T.G., Vaughan, D.G. and Corr, H.F.J. 2006. New boundary conditions for the West Antarctic Ice Sheet: Subglacial topography of the Thwaites and Smith glacier catchments, Geophys. Res. Lett., 33, L09502, doi:10.1029/2005GL025561.
- ↑ Vaughan, D.G., Corr, H.F.J., Ferraccioli, F., Frearson, N., O'hare, A., Mach, D., Holt, J.W., Blankenship, D.D., Morse, D.L. and Young, D.A. 2006. New boundary conditions for the West Antarctic ice sheet: Subglacial topography beneath Pine Island Glacier, Geophys. Res. Lett., 33, L09501, doi:10.1029/2005GL025588.
- ↑ Naruse, R. 1979. Thinning of the ice sheet in Mizuho Plateau, East Antarctica, J. Glaciol., 24, 45-52.
- ↑ King, M.A. 2004. Rigorous GPS data-processing strategies for glaciological. Applications, J. Glaciol., 50, 601-607.
- ↑ Scambos, T.A., Dutkiewicz, M.J., Wilson, J.C. and Bindschadler, R.A. 1992. Application of image cross-correlation to the measurement of glacier velocity using satellite image data, Remote Sensing of Environment, 42, 177-186.
- ↑ Pritchard, H.D. and Vaughan, D.G. 2007. Widespread acceleration of tidewater glaciers on the Antarctic Peninsula, J. Geophys. Res., 112, F03S29, doi:10.1029/2006JF000597.
- ↑ Gray, L.; Short, N.; Bindschadler, R.; Joughin, I.; Padman, L.; Vornberger, P.; Khananian, A. 2002. Radarsat interferometry for Antarctic grounding-zone mapping, Annals of Glaciology, 34, 269-276.
- ↑ Yamanokuchi, T., Doi, K. and Shibuya, K. 2005. Validation of grounding line of the East Antarctic Ice Sheet derived by ERS-1/2 interferometric SAR data, Polar Geoscience, 18, 1-14.
- ↑ 26.0 26.1 Rignot, E. and Thomas, R.H. 2002. Mass balance of polar ice sheets, Science, 297 (5586), 1502-1506 AUG 30 2002.
- ↑ Fischer, A.H., Rott, H. and Björnsson, H., 2003. Observation of recent surges of Vatnajökull, Iceland, by means of ERS SAR interferometry, Annals of Glaciology, 37, 69-76.
- ↑ Fricker, H.A., Young, N.W., Allison, I. and Coleman, R. 2002. Iceberg calving of the Amery Ice Shelf, East Antarctica: New field and remote sensing data, Annals of Glaciology, 34, 81-88.
- ↑ Nakamura K., Doi, K. and Shibuya, K. 2007. Estimation of seasonal changes in the flow of Shirase Glacier using JERS-1/SAR image correlation, Polar Sci., 1(2-4), 73-84.
- ↑ Bindschadler, R. A., King, M.A., Alley, R.B., Anandakrishnan, S. and Padman, L. 2003, Tidally controlled stick-slip discharge of a West Antarctic ice stream, Science, 301, 1087-1089.
- ↑ Gudmundsson, G.H. 2006. Fortnightly variations in the flow velocity of Rutford Ice Stream, West Antarctica, Nature, 444, 1063-1064 doi:10.1038/nature05430.
- ↑ Jezek, K. C. 2008. The RADARSAT-1 Antarctic Mapping Project. BPRC Report No. 22, Byrd Polar Research Center, The Ohio State University, Columbus, Ohio, 64 p.
- ↑ Arthern, R.J. and Hindmarsh, R.C.A. 2006. Determining the contribution of Antarctica to sea-level rise using data assimilation methods, Phil. Trans. R. Soc. A, 364, 1841-1865. doi:10:1098/rsta.2006.1801.
- ↑ Fricker, H., Warner, R. and Allison, I. 2000. Mass balance of the Lambert Glacier–Amery Ice shelf system, East Antarctica: a comparison of computed balance fluxes and measured fluxes, J. Glaciol., 46 (155), 561-570.
- ↑ Smith, A.M., Vaughan, D.G., Doake, C.S.M., ET AL. 1998. Surface lowering of the ice ramp at Rothera Point, Antarctic Peninsula, in response to regional climate change, Ann. Glaciol., 27, 113-118.
- ↑ 36.0 36.1 Hamilton, G.S. 2005. Spatial patterns in mass balance of the Siple Coast and Amundsen Sea sectors, West Antarctica, Ann. Glaciol., 41, 105-106.
- ↑ Wendt, A., G. Casassa, A. Rivera, and J. Wendt (2009). Reassessment of ice mass balance at Horseshoe Valley, Antarctica. Antarctic Science. doi:10.1017/S0954102009002053.
- ↑ Thomas, R.H. 1973. The creep of ice shelves: Theory, Journal of Glaciology, 12 (64), 45-53.
- ↑ Walker, R. and Holland, D.M. 2007. A two-dimensional coupled model for ice shelf - ocean interaction, Ocean Modelling, 17, 123-139.
- ↑ Rist, M.A., Sammonds, P.R., Oerter, H. and Doake, C.S.M. 2002. Fracture of Antarctic shelf ice, J. Geophys. Res., 107(B1), 10.1029/2000JB000058.
- ↑ Nicholls, K.W. and Jenkins, A. 1993, Temperature and salinity beneath Ronne Ice Shelf, Antarctica, J. Geophys. Res., 98, 22,553-22,568.
- ↑ Craven, M., Allison, I., Brand, R., Elcheikh, A., Hunter, J., Hemer, M. and Donoghue, S. 2004. Initial borehole results from the Amery Ice Shelf hot-water drilling project, Ann. Glaciol., 39, 531-539.
- ↑ Corr, H.F.J., Jenkins, A., Nicholls, K.W. and Doake, C.S.M. 2002. Precise measurement of changes in ice-shelf thickness by phase-sensitive radar to determine basal melt rates, Geophys. Res. Lett., 29(8), 10.1029/2001GL014618.
- ↑ Jenkins, A., Corr, H.F.J., Nicholls, K.W., Stewart, C.L., Doake, C.S.M. and Christopher S.M. 2006. Interactions between ice and ocean observed with phase-sensitive radar near an Antarctic ice-shelf grounding line, J. Glaciol., 52 (178), 325-346.
- ↑ King, M.A., Coleman, R., Morgan, P.J. and Hurd, R.S. 2007. Velocity change of the Amery Ice Shelf, East Antarctica, during the period 1968-1999, J. Geophys. Res., 112, F01013, doi:10.1029/2006JF000609.
- ↑ Vaughan, D.G. 1995, Tidal Flexure at Ice Sheet Margins, Journal of Geophysical Research, 100 (B4), 6213-6224.
- ↑ Padman, L. and Fricker, H.A. 2005. Tides on the Ross Ice Shelf observed with ICESat, Geophysical Research Letters, 32, No. 14, Art. No. L14503, July 29, 2005.
- ↑ 48.0 48.1 Raymond, C.F., Echelmeyer, K.A., Whillans, I.M. and Doake, C.S.M. 2001. Ice stream shear margins. In: The West Antarctic Ice Sheet: Behavior and environment (R.B. Alley and R.A. Bindschadler, eds.). Washington DC: American Geophysical Union, Antarctic Research Series, 77, 137-155.
- ↑ Retzlaff, R. and Bentley, C.R. 1993. Timing of stagnation of Ice Stream C, West Antarctica, from short-pulse radar studies of buried surface crevasses, Journal of Glaciology, 39, No. 133, 553-561.
- ↑ 50.0 50.1 Stokes, C.R., Clark, C.D., Lian, O.B. and Tulaczyk, S. 2007. Ice stream sticky spots: A review of their identification and influence beneath contemporary and palaeo-ice streams, Earth-Science Reviews, 81, 217-249.
- ↑ Anandakrishnan, S. and Alley, R.B. 1994. Ice stream C, Antarctica, sticky spots detected by microearthquake monitoring, Ann. Glaciol., 20, 183-186.
- ↑ Smith, A.M. 2007. Subglacial Bed Properties from Normal-Incidence Seismic Reflection Data, J. Env. and Eng. Geophys., 12, 3-13.
- ↑ Carsey, F., Behar, A., Lane, A.L., Realmuto, V. and Engelhardt, H. 2002. A borehole camera system for imaging the deep interior of ice sheets, J. Glaciol., 48 (163), 622-628.
- ↑ 54.0 54.1 Vogel, S.W., Tulaczyk, S., Kamb, B., Engelhardt, H., Carsey, F., Behar, A., Lane, A. and Joughin, I. 2005. Subglacial Conditions During And After Stoppage of an Antarctic Ice Stream: Is Reactivation Imminent, Geophys. Res. Lett., 32 (14), L14502, doi:10.1029/2005GL022563.
- ↑ Christoffersen, P. and Tulaczyk, S. 2003. Response of subglacial sediments to basal freeze-on, 1. Theory and comparison to observations from beneath the West Antarctic Ice Sheet, J. Geophys. Res., 108(B4), 2222, doi:10.1029/2002JB001935.
- ↑ Alley, R.B., Anandakrishnan, S., Bentley, C.R. and Lord, N. 1994, A water-piracy hypothesis for the stagnation of Ice Stream C, Antarctica. Ann. Glaciol., 20, 187-194.
- ↑ Gray, L., Joughin, I., Tulazcyk, S., Spikes, V.B., Bindschadler, R. and Jezek, K. 2005. Evidence for subglacial water transport in the West Antarctica Ice Sheet through three-dimensional satellite radar interferometry, Geophysical Research Letters, 32, L03501, doi:10.1029/2004GL021387,.
- ↑ Wingham, D.J., Siegert, M.J., Shepherd, A. and Muir, A.S. 2006b. Rapid discharge connects Antarctic subglacial lakes, Nature, 440, pp. 1033-1036 , doi:10.1038/nature04660.
- ↑ Fricker, H.A., Scambos, T.A., Bindschadler, R. and Padman, L. 2007. An Active Subglacial Water System in West Antarctica Mapped from Space, Science, 315, 1544, doi: 10.1126/science.1136897.
- ↑ Alley, R.B. and R.A. Bindschadler. 2001, The West Antarctic Ice Sheet: Behavior and environment. Washington DC: American Geophysical Union, Antarctic Research Series vol. 77, 296 pp.
- ↑ Bindschadler, R. 2006. The environment and evolution of the West Antarctic ice sheet: setting the stage, Phil. Trans. R. Soc. A, 364, 1583-1605, doi:10.1098/rsta.2006.1790.
- ↑ Monaghan, A.J., Bromwich, D.H. and Wang, S-H. 2006a. Recent trends in Antarctic snow accumulation from Polar MM5, Philosophical Trans. Royal. Soc. A, 364, 1683-1708.
- ↑ 63.0 63.1 Richardson, C. and Holmlund, P. 1999. Regional and local variability in shallow snow layer depth from a 500 km continuous radar traverse on the polar plateau, central Dronning Maud Land, East Antarctica, Annals of Glaciology, 29, 10-16.
- ↑ 64.0 64.1 64.2 64.3 Frezzotti, M., Pourchet, M., Flora, O., Gandolfi, S., Gay, M., Urbini, S., Vincent, C., Becagli, S., Gragnani, R., Proposito, M., Severi, M., Traversi, R., Udisti, R. and Fily, M. 2004. New estimations of precipitation and surface sublimation in East Antarctica from snow accumulation measurements, Climate Dynamics, 23, 803-813.
- ↑ 65.0 65.1 Spikes, V.B., Hamilton, G.S., Arcone, S.A., Kaspari, S. and Mayewski, P.A. 2004. Variability in accumulation rates from GPR profiling on the West Antarctic Plateau, Ann. Glaciol., 39, 238-244.
- ↑ Nishio, F., Furukawa, T., Hashida, G., Igarashi, M., Kameda, T., Kohno, M., Motoyama, H., Naoki, K., Satow, K., Suzuki, K., Takata, M., Toyama, Y., Yamada, T. and Watanabe, O. 2002. Annual-layer determinations and 167 year records of past climate of H72 ice core in east Dronning Maud Land, Antarcitaca, Ann. Glacio., 35, 471-479.
- ↑ Oerter, H., Graf, W., Wilhelms, F., Minikin, A. and Miller, H. 1999. Accumulation studies on Amundsenisen, Dronning Maud Land, Antarctica, by means of tritium, dielectric profiling and stable-isotope measurements: first results from the 1995-96 and 1996-97 field seasons, Ann. Glaciol., 29, 1-9.
- ↑ Magand, O., Frezzotti, M., Pourchet, M., Stenni, B., Genoni, L. and Fily, M. 2004. Climate variability along latitudinal and longitudinal transects in East Antarctica, Annals of Glaciology, 39, 351-358.
- ↑ 69.0 69.1 Rotschky, G., Eisen, O., Wilhelms, F., Nixdorf, U. and Oerter, H. 2004. Spatial distribution of surface mass balance on Amundsenisen plateau, Antarctica, derived from ice-penetrating radar studies, Annals of Glaciology, 39, 265-270.
- ↑ Urbini, S., Gandolfi, S. and Vittuari, L. 2001. GPR and GPS data integration: examples of application in Antarctica, Annali di Geofisca, 44 (4), 687-702.
- ↑ Arcone, S.A., Spikes, V.B., Hamilton, G. and Mayewski, P.A. 2005. Continuity, vertical resolution and origin of stratigraphy in 400-Mhz short-pulse radar profiles of firn in West Antarctica, Annals of Glaciology, 39, 195-200.